div epub:type=”chapter” role=”doc-chapter”>
S. Stübinger et al. (eds.)Lasers in Oral and Maxillofacial Surgeryhttps://doi.org/10.1007/978-3-030-29604-9_9
9. Laser in Bone Surgery
Abstract
During the past half-century, laser osteotomy has been studied for a broad range of lasers, which almost covers the entire range of available laser systems in the market, from early unsuccessful experiments with CW lasers to newly developed ultrashort pulse lasers. Although a large variety of laser parameters including wavelength, pulse energy, pulse duration, and repetition rate have been investigated to find an optimum laser system as an alternative osteotomy tool, there is not a universal agreement on a specific type of laser to replace conventional mechanical saws. The only universal agreement is on the speed of cutting (ablation rate) which went to long-pulse Er:YAG and CO2 lasers. Microsecond pulse Er:YAG and CO2 lasers perform osteotomy by inducing efficient photothermal effect to the bone with the help of high absorption peak of water in the bone. However, having a speedy cut is not the only effective parameter to pave the way for transferring lasers to the operating room. Other parameters including cutting with the lowest thermal damage, ability for deep cutting, and compatibility with integrating sensors are among the other determinant parameters. Moreover, being able to be delivered through the fiber optic and as a consequence fit inside the endoscope channel could extend their application from the open surgery to minimally invasive ones. This chapter besides proving the necessary information on the physics behind the laser–bone interaction provides a short review on the history of bone surgery with laser and state-of-the-art studies in this field.
Laser osteotomyBone cuttingHard boneEr:YAGCO2Nd:YAGIrrigationCarbonizationAblation efficiencyPulse duration
9.1 Introduction
Bone surgery has been a challenge since ancient times due to the difficulties for performing free and precise shapes and trying to preserve, at the same time, the surrounding tissues. However, the conventional tools have not changed according to the new challenges and needs in the operating rooms [1]. The surgeons still are feeling more comfortable using the classical mechanical saws and drills despite the collateral effects those procedures have on the patients. The vibrations and heat generated by those mechanical tools provoke damage of soft tissue and delayed healing process for the cut area [2].
The main areas in which laser technology has achieved the best results in terms of less damage, more flexibility to perform the cuts, and better healing process for the patient are dermatology [3], ophthalmology [4], otolaryngology [5], and dentistry [6]. All those areas are mainly focused in soft tissue rather than in hard tissue. The only clinical application in which lasers like erbium, Nd:YAG, and CO2 have been used to ablate hard tissue, like enamel and cementum, is in dentistry [7]. However, no deep cuts have been yet achieved. In the last decades, the use of laser for ablating oral tissues has shown a better performance because the laser provides small and flexible cuts and contact-free interaction with the cut area. Therefore, the collateral damage is reduced [7–10], and the healing process is much faster and easier for the patient.
9.2 History of Hard Tissue Laser Ablation
Bone surgery is found to be more challenging when fragile, and small bone parts (like in the maxilla and mandible) are under high pressure and vibration due to the contact with the mechanical tools (osteotomes). Also, the difficulties are found when cutting bones which are surrounded by delicate tissue like the spine or bones which require an invasive surgery to be reached, like in the partial or complete replacement of the knee. All those procedures require precision, less contact pressure and vibrations, and free shape cuts. Additionally, it is needed to reduce the bacterial contamination risk, which is very high when using the conventional osteotomes like drills and saws because sometimes there is deposition of metal material in the cut areas [7].
Right after the invention of the laser by Maiman in 1960 [11], many more types of lasers were launched [12]. Moreover, pulsed lasers were studied as potential tools in biomedical applications [13, 14], and only 5 years after its invention, the laser was used for precise tissue coagulation [15].
On the contrary, laser ablation of hard tissue was also investigated as early as other biomedical applications. The first studies were performed by hitting molar and incisor teeth using a ruby laser at the wavelength λ = 694.3 nm [16]. In 1964, the Nd:YAG and the CO2 lasers were developed at the Bell Telephone Laboratories [17, 18]. Those lasers have been mostly used in dentistry applications for ablating soft and hard tissue, especially the CO2 laser has been highly used since then. However, the ablation processes using the CO2 laser showed that the tissues were getting carbonized due to the action of the laser [19, 20], and these carbonization effects led to delayed bone healing in comparison with the effect of the conventional mechanical tools. Even when changing the laser parameters, similar results were obtained [21, 22]. Studies of temperature rise and its effects while ablating the bone [23] showed that temperatures between 44 °C and 47 °C are critical and the consequences are the tissue presents thermal damage, which makes the healing process much more difficult, and, in some cases, the complete death of tissue in the surroundings of the ablated area.
The first studies in bone ablation using a pulsed Er:YAG laser were performed by Nuss [24] in 1988 and Hibst and Keller [25, 26] in 1989, where the interaction of middle-infrared wavelength laser with hard tissue was starting to be understood. Ten years after, deeper research in the interaction of the laser with the hard tissues showed that the most efficient wavelengths for ablating bone were 2.9, 3.0, and 5.9–6.45 μm [27]. When additional and proper amount of water spray was used together with the erbium laser to ablate the bones, the thermal damage was dramatically reduced [28]. In general, erbium laser wavelengths like 2.94 μm from the Er:YAG laser and 2.78 μm from the Er,Cr:YSGG laser were used and proven to be very efficient for dental hard tissue ablation [29, 30]. Nowadays, the lasers which are mainly used for hard tissue ablation are the Nd:YAG, the CO2, and the erbium lasers.
9.3 The Physics Behind the Laser–Bone Interaction
The tissue properties involved in the interaction with the laser are absorption, scattering, anisotropy, and refractive index. The properties of the laser irradiation which might affect the tissues are wavelength, exposure time, pulse frequency, pulse duration, spot size, power, and energy density. There are two main types of laser–tissue interaction: Once the laser impinges the tissue, the light can be affected by the tissue properties, or the tissue can be affected by the laser parameters. In both cases, the laser parameters affect the result as well as the tissue properties. The first type of interaction is related to the field of diagnosis, imaging, and spectroscopy. All those methods are used basically to understand what is happening inside the tissues, what are their components, etc., by measuring the light properties after the interaction with the tissues. In the second type, the light affects the tissues because it is changing their properties; the laser can be used for surgical and therapeutic ablation, coagulating, and welding. Here we are interested in the second type. When the tissues are affected by the laser light, three main phenomena can occur: photochemical, photothermal, or photomechanical interactions [31]. Photodynamic therapy is a well-known procedure in which the photochemical interaction takes part. In this process, a photon interacts with a photosensitive dye and oxygen; from this reaction, a toxic material is produced, and therefore, the cancer cells are killed [31]. The photothermal interaction is the vaporization of tissue caused when the tissue absorbs the laser energy [32]. In the photomechanical interaction, the tissue is expelled, for instance, by explosions or the formation of plasma, in which the absorption properties of the tissues are not the main causes of tissue ablation [33]. In the following sections, we discuss the two main mechanisms used to ablate hard tissue: photothermal and photomechanical ablation.
Along the history of hard tissue laser ablation, the lasers which have been more used are the Nd:YAG, Ho:YAG, Er,Cr:YSGG, Er:YAG, CO2, and ultrashort pulsed lasers. In the following, we discuss the ablation process driven by the interaction of those lasers and the hard tissues.
9.3.1 The Middle-Infrared Lasers
9.3.1.1 The Effect of Water Absorption
Water has the highest peaks of absorption at the middle-infrared wavelengths (2–10 μm) [33, 34]; this is the key point of working principle of the middle-infrared lasers to ablate the tissues better compared to other wavelengths, but that is not the only reason. In the case of hard tissues like enamel, cementum, dentin, and bone, the ablation process is driven by a thermomechanical event, which involves photothermal and photomechanical ablation [35, 36]. When the laser impinges the hard tissue surface, the energy is absorbed by the tissue and transferred into heat energy to the interstitial matrix inside the bone, the heat yields very high pressures in the water molecules of the tissue, and therefore, the ejection of the tissue occurs by explosive vaporization [7, 36, 37]. Therefore, the higher the absorption of water, the faster the ablation is [27], and this is the reason why under proper water cooling conditions, the Er:YAG laser working at λ = 2.94 μm (water has the highest absorption in the spectrum [33, 34]) is up to now the best laser known which is able to ablate bone very efficiently [36, 38–42]. Studies have shown that the Er:YAG working at the wavelength 2.94 μm is more efficient in ablating hard tissue at higher speeds compared to Er,Cr:YSGG working at 2.78 μm [40, 43], and the main reason is that the absorption coefficient of Er:YAG is 1200 mm−1, three times more than the one for Er,Cr:YSGG (400 mm−1). The Ho:YAG laser has been also used to ablate bone; however, it has shown low ablation efficiency compared to Er:YAG lasers. The Ho:YAG laser works at the wavelength λ = 2.1 μm, in which water has ca. 120 times less absorption (10 mm−1); this explains why this laser is not sufficiently efficient for bone ablation [44]. The CO2 laser works at the wavelength 10.6 μm; the water absorption coefficient is 79 mm−1 [33, 45], approximately 15 times lower than the water absorption at Er:YAG wavelength. This laser has also been used for ablating hard and soft tissue, and the results show that its ablation efficiency is also very low in comparison with Er:YAG laser [46, 47].
9.3.1.2 The Effect of Pulse Duration
![$$ {D}_{\mathrm{d}}=\left(1/\alpha \right)\ln \left[{F}_{\mathrm{th}}\alpha /\left({T}_{\mathrm{c}}-{T}_0\right){\rho}_{\mathrm{c}}\right], $$](https://i0.wp.com/pocketdentistry.com/wp-content/uploads/2021/07/435642_1_En_9_Chapter_TeX_Equa.png?w=960)
where α is the absorption coefficient of the material at the specific wavelength of the laser used, Fth is the threshold fluence for ablation, Tc is the critical temperature for the denaturation of the tissue, T0 is the initial tissue temperature before irradiation, and ρc is the volumetric-specific heat of the tissue [46].

The results reported in [48] using a Q-switched Er:YAG for ablating pig skin, aorta, and bone give damaged widths bigger than the predicted by the first model and smaller than the predicted by the second model. In that study, they show that the damage created by ns laser pulses is less than for the μs laser pulses. Similar results are shown when using a CO2 laser [46].
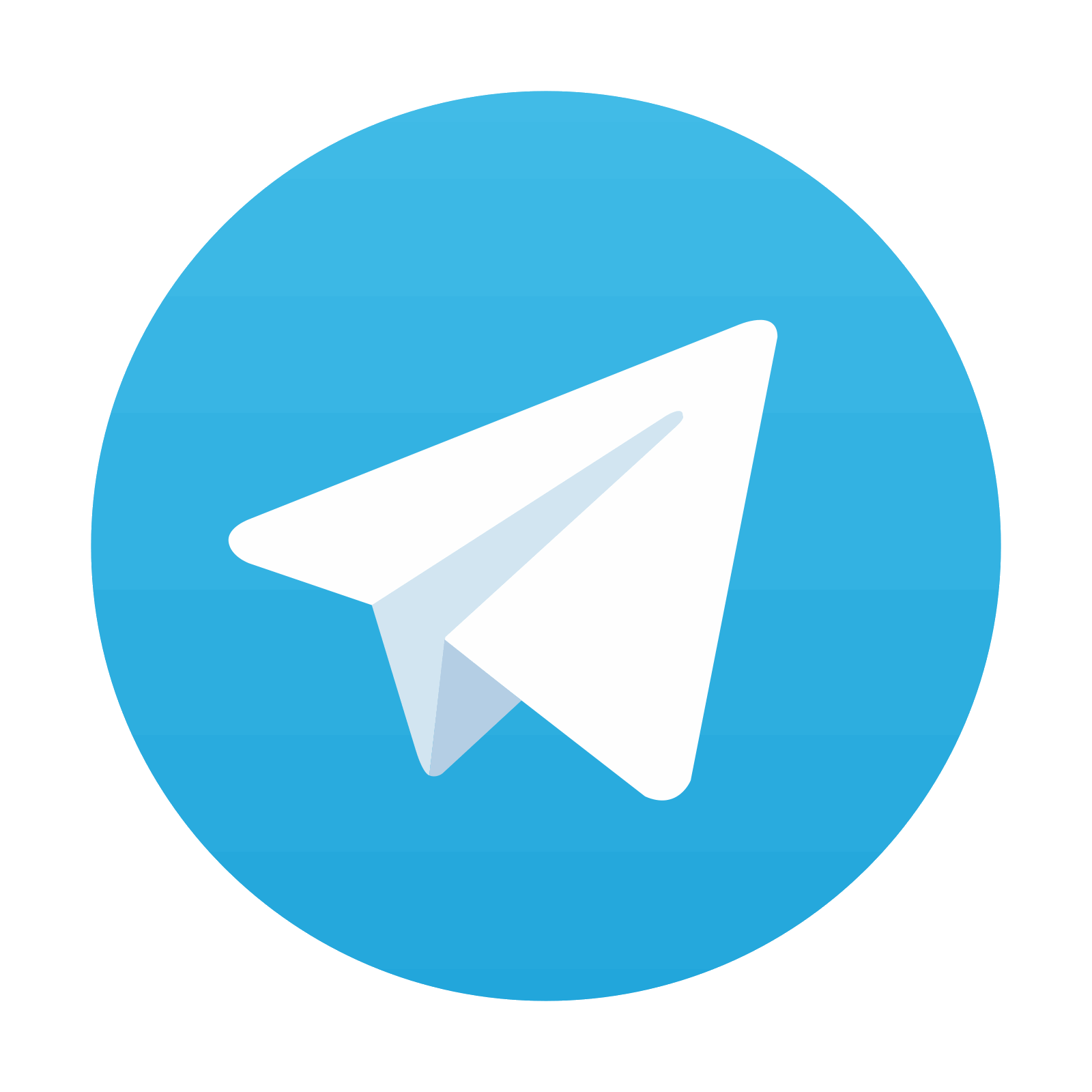
Stay updated, free dental videos. Join our Telegram channel

VIDEdental - Online dental courses
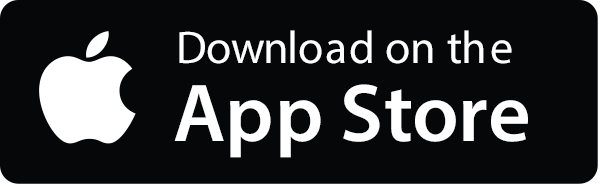
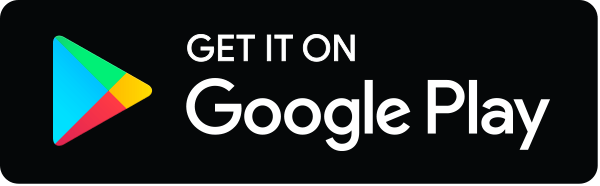