Abstract
Introduction
Periodontal ligament (PDL) damage caused by dental trauma can lead to local circulatory disorders. The mechanisms through which PDL cells, once exposed to a transient hypoxic environment, contribute to tissue regeneration or resorption of pathological tooth roots after reoxygenation remain unclear. Therefore, we aimed to examine how changes in oxygen (O 2 ) concentration affect PDL healing.
Materials and methods
Human PDL stem cells (hPDL cells) were cultured under normoxic or hypoxic (20% or 1% O 2 concentration) conditions. Vascular endothelial growth factor (VEGF) and receptor activator of nuclear factor kappa-Β ligand (RANKL) expressions were measured using real-time quantitative polymerase chain reaction or Western blotting. Furthermore, a co-culture of hPDL and osteoclast precursor cells was used to demonstrate the effect of changes in O 2 concentration on osteoclast formation.
Results
VEGF expression considerably increased over time under hypoxia compared with normoxia. However, during reoxygenation (24 h hypoxia–24 h normoxia), expression markedly decreased under hypoxia. No significant difference in RANKL expression was observed in both conditions after 24 h; however, it remarkably increased under hypoxia compared with normoxia after 48 h. In the osteoclast formation assay, the number of tartrate-resistant acid phosphatase (TRAP)-positive multinucleated cells considerably increased over time under hypoxia compared with normoxia. Notably, when VEGF expression was reduced using small interfering RNA, the number of TRAP-positive multinucleated cells decreased extensively.
Conclusion
Under hypoxic conditions, periodontal ligament cells produce VEGF to promote angiogenesis. However, excessive VEGF production, along with RANKL production, induces osteoclast formation. Osteoclast formation can be suppressed using rapid reoxygenation.
1
Introduction
The periodontal ligament (PDL) anchors the tooth within the alveolar bone and maintains homeostasis of the periodontal tissue [ ]. The PDL contains nerves and serves as a sensory organ that senses various external stimuli. This ligament also contains various cells, including fibroblasts, osteoblasts, cementoblasts, and epithelial remnants of Malassez [ ]. Seo et al. reported the presence of stem cells in the PDL. Stem cells can differentiate into fibroblasts and osteoblasts and contain capillary tissue essential for maintaining PDL homeostasis [ , ]. Because the PDL is constantly exposed to mechanical stresses, fibroblasts in the PDL have considerably higher collagen synthesis and degradation ability and higher metabolic activity than those in other skin and gingiva [ ]. Therefore, to maintain activity of fibroblasts in the PDL, abundant oxygen (O 2 ) is constantly supplied from the vascular network in the PDL. The vasculature of the PDL plays a crucial role in maintaining homeostasis. However, in the oral cavity, PDL becomes hypoxic under certain conditions. For instance, excessive occlusal forces, such as occlusal trauma or tooth movement due to orthodontic treatment, have been reported to compress the PDL, reduce blood flow, and create a hypoxic environment in the PDL [ ]. Hypoxia stimulates expression of bioactive substances in various cells. Therefore, the mechanism through which cytokines are induced by a hypoxic environment and maintenance of homeostasis in the body are debatable. Generally, during hypoxia, angiogenesis is enhanced as a compensatory measure to maintain homeostasis, and O 2 supply is improved through increased number of blood vessels [ ]. In this case, the newly formed blood vessels in the tissue are functional blood vessels with blood flow. At the cellular level, hypoxia induces angiogenic factors, such as vascular endothelial growth factor (VEGF), through hypoxia-inducible factor (HIF) [ , ]. Moreover, hypoxic environments have been shown to induce angiogenic factors, such as VEGF, in PDL cells [ ]. Therefore, angiogenesis is crucial in the early stages of tissue healing; if angiogenesis is hindered during these stages, healing is impaired. Therefore, production of angiogenic factors, such as VEGF, plays an essential role in damaged tissues. When a tooth dislodges or invaginates due to trauma, capillaries in the PDL rupture, leading to local circulatory disorders at the rupture site. This exposes the cells constituting the PDL to hypoxia due to ischemia. However, if appropriate treatments, such as reduction and immobilization, are administered, restoration of blood circulation reoxygenates the tissue [ ]. The healing process of PDL after trauma is complex. The mechanisms through which PDL cells, once exposed to a transient hypoxic environment, contribute to tissue regeneration or resorption of pathological tooth roots after reoxygenation remain unclear. Therefore, in the present study, we aimed to examine how changes in O 2 concentration affect PDL regeneration by performing an in vitro analysis. We used human PDL stem ligament cells (hPDL cells) to simulate hypoxic exposure and subsequent reoxygenation.
2
Materials and Methods
2.1
Cell line and culture
The hPDL cells were obtained from Tokushima University (Tokushima, Japan) [ , ]. They were cultured in minimal essential medium Eagle–alpha modification (α-MEM) supplemented with 10% fetal bovine serum (FBS; Thermo Fisher Scientific, Waltham, MA, USA) and 60 μg/mL of kanamycin at 37 °C in 95% air and 5% carbon dioxide (CO 2 ). Hypoxia was induced using Anero pack Kenki (Mitsubishi Gas Chemical, Tokyo, Japan), a disposable O 2 absorbing and CO 2 generating agent that reduces O 2 concentration to 1% and maintains CO 2 concentration at approximately 5%. Three experimental groups were created: Group 1: Normaxia (hPDL cells were incubated at 20% O 2 and 5% CO 2 at 37 °C for 24 or 48 h in a CO 2 incubator); Group 2: Hypoxia (hPDL cells were incubated at 1% O 2 and 5% CO 2 at 37 °C for 24 or 48 h using Anero pack Kenki); Group 3: Reoxygenation (hPDL cells were first cultured under hypoxia for 24 h, then under normoxia for 24 h).
2.2
Real-time quantitative polymerase chain reaction analysis
VEGF and receptor activator of nuclear factor kappa-Β ligand (RANKL) messenger RNA (mRNA) expressions were measured using real-time quantitative polymerase chain reaction (RT-qPCR). RNA quality and concentration were analyzed using a NanoDrop 2000 spectrophotometer (Thermo Fisher Scientific). Purified total RNA was converted into complementary DNA using a recombinant RNase Inhibitor (TaKaRa, Tokyo, Japan), PrimeScript™ Reverse Transcriptase (TaKaRa), deoxynucleotide triphosphate Mixture (TaKaRa), and random primers (Invitrogen, Carlsbad, CA, USA). RT-qPCR assays were performed using SYBR Premix Ex Taq II (TaKaRa), and data were analyzed using a Thermal Cycler Dice Real-Time System TP800 (TaKaRa). For mRNA quantification, target expression levels were normalized to β-actin mRNA levels. Primer sequences used for RT-qPCR are listed in Table 1 .
Gene | Sequences (F: forward, R: reverse) | GenBank number |
---|---|---|
VEGF | F 5′ TCA CAG GTA CAG GGA TGA GGA CAC 3′ | NM_001025366.3 |
R 5′ CAA AGC ACA GCA ATG TCC TGA AG 3′ | ||
RANKL | F 5′ GCC TTT CAA GGA GCT GTG CAA AA 3′ | NM_003701 |
R 5′ GAG CAA AAG GCT GAG CTT CAA GC 3′ | ||
β-actin | F 5′ TGG CAG CCA GCA CAA TGA a 3′ | NM_001101.4 |
R 5′ CTA AGT CAT AGT CCG CCT AGA AGC a 3′ |
2.3
Western blotting
Cells were harvested and lysed using the radioimmunoprecipitation assay buffer (FUJIFILM Wako, Tokyo, Japan). Protein concentration was determined using Quick Start Bovine Serum Albumin Standard (Bio-Rad, Hercules, CA, USA). Proteins in cell lysates were separated using electrophoresis on Novex 10–20% Tricine Protein Gels (Thermo Fisher Scientific). Separated proteins were transferred to Immobileon polyvinylidene fluoride/filter sandwiches (Thermo Fisher Scientific) and blocked with 5% Blocking One (NACALAI TESQUE, Kyoto, Japan) for 30 min. The membrane was incubated with rabbit anti-VEGF and anti–HIF–1α antibodies ( × 1000) (Novus Biologicals, Littleton, CO, USA) or rabbit anti-β-actin antibody ( × 1000) (Gene Tex, Irvine, CA, USA) overnight at 4 °C, followed by incubation with goat anti-rabbit Immunoglobulin G (H + L) secondary antibody ( × 10,000) (Bio-Rad) for 2 h at room temperature. Western Enhanced Chemiluminescence Substrate (Bio-Rad Laboratories) was used to detect specific protein bands on membranes.
2.4
Co-culture experiments
The hPDL cells (1.0 × 10 4 cells/well) were seeded in a 96-well plate to investigate osteoclast formation. After confirming confluence of the hPDL cells, they were cultured under hypoxia for 24 h or 48 h, normoxia for 24 h or 48 h, and reoxygenation (hypoxia 24 h–normoxia 24 h). They were then fixed with formalin and washed thrice with phosphate-buffered saline (−). Next, human osteoclast precursor cells (3.0 × 10 4 cells/well) were seeded onto the hPDL cells using fresh α-MEM supplemented with 10% FBS and 1.75 μM of macrophage colony-stimulating factor. Human osteoclast precursor cells, derived from human bone marrow mononuclear cells, were purchased from COSMO BIO (Tokyo, Japan).
After 1 week of co-culture at 37 °C in 95% air and 5% CO 2 , cells were fixed and stained using a tartrate-resistant acid phosphatase (TRAP) Staining Kit (COSMO BIO) according to the manufacturer’s instructions. TRAP-positive multinucleated cells containing at least three nuclei were counted as osteoclasts under a light microscope (Keyence, Osaka, Japan).
2.5
Small interfering RNA transfection
To suppress VEGF expression in hPDL cells, a predesigned small interfering RNA (siRNA; siRNA ID: HSS111275; Thermo Fisher Scientific) was introduced using forward transfection. SignalSilence Control siRNA (Unconjugated) (Thermo Fisher Scientific) was used as a negative control. A mixture containing Opti-Mem-I Reduced Serum Medium, Lipofectamine RNAIMAX REAGENT (Thermo Fisher Scientific), and siRNA was added to hPDL cells and cultured under the abovementioned conditions.
2.6
Statistical analysis
All data were presented as mean ± standard error. Statistical significance was determined using Student’s t-test or Mann–Whitney U test for pairwise comparisons and Bonferroni’s multiple comparison test for multiple comparisons. Statistical significance was set at P < 0.05.
3
Results
3.1
VEGF and HIF-1α expressions in hPDL cells exposed to hypoxia
HIF-1α protein expression in hPDL cells exposed to normoxia or hypoxia for 24 h was assessed. Western blotting revealed that VEGF and HIF-1α expressions in hPDL cells considerably increased under hypoxia compared with normoxia ( Fig. 1 , Appendix 1 ).
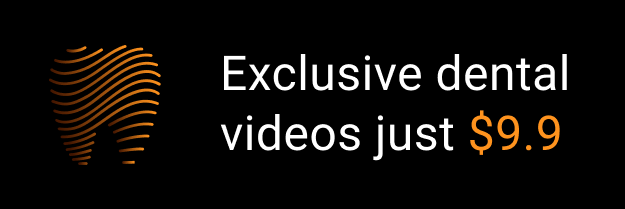