Riva Touger-Decker, Connie Mobley and Joel B. Epstein (eds.)Nutrition and HealthNutrition and Oral Medicine2nd ed. 201410.1007/978-1-60761-490-6_10
© Springer Science+Business Media New York 2014
10. Genomics and Oral Health: An Overview
(1)
DeBusk Communications, LC, 3583 Doris Drive, Tallahassee, FL 32303-2304, USA
Keypoints
-
Alterations to DNA that may occur include changes in chromosomal number or structure, changes in DNA sequence, and changes in the epigenome
-
In oral health and disease, detection of genetic alterations can be used for a wide spectrum of applications, from distinguishing pathologically similar disorders to predicting susceptibility to various oral diseases, such as caries, periodontal disease, and oral cancers
-
Pharmacogenomics and nutritional genomics are new approaches to diagnosing oral diseases, profiling patients’ gene-directed drug-metabolizing capabilities, determining dietary requirements based on genotype, and making gene-directed food choices
-
Nutritional genomics is a specialized application of genomics to diet- and lifestyle-related disease, which includes the majority of the chronic disorders of modern societies
Keywords
GenomicsOral healthOral health and diseaseGenetic alterationsNutritional genomicsPharmacogenomics
Introduction
The Human Genome Project is credited with the arrival of the genomics era. The project was initiated in 1990 and achieved its primary goals by 2003. A multinational collaboration, the project’s initial goal was to identify each of the 3 billion DNA nucleotides of the human genetic material and to estimate the number of genes. Although this project has been completed, the core exploration has continued with an expanded focus that includes identifying the function of each gene and its protein product, dissecting the complexity of gene expression and its regulation, and defining the genomes of other organisms used as model systems to help decipher the workings of the human genome. Further, several other important “-omics” disciplines have emerged from the Human Genome Project, such as transcriptomics, proteomics, and metabolomics. Each is focused on understanding key aspects of how the information stored within an organism’s genome is converted into the complicated cellular machinery that sustains life.
Additional “-omics” disciplines, such as epigenomics, pharmacogenomics, nutritional genomics, and microbiomics, focus on how factors from the environment that an organism inhabits communicate with the genetic material to impact the ultimate biological outcome. The weblike complexity of higher organism functioning can be daunting to dissect but, fortunately, the blueprint for life is fundamentally the same among organisms so that many of the findings from research with less complex organisms can be extrapolated to human implications. An overview of the Human Genome Project can be found at the websites for the National Human Genome Research Institute (www.genome.gov) and the U.S. Department of Energy (genomics.energy.gov), which co-administered this global project.
The Human Genome Project brought to the forefront of healthcare an important fundamental biological principle: genes underlie function. There is a direct connection between the information in our genes and our ability to carry out the myriad activities that support life. This awareness alters our thinking about dysfunction (“disease”) because it’s now clear that there is a logical underpinning to disease, one that can be systematically dissected and targeted with appropriate therapeutic interventions. We do not “get” a disease; disease develops because our individual genetic makeup includes gene variants (also called “genetic variations”) that make us susceptible to disease through their ultimate influence on physiological function. The susceptibility may be strong or it may be too weak in itself to lead to significant dysfunction unless one or more environmental factors interacts with those variants. The chronic diseases that challenge healthcare today are the result of interactions between our genes and our environment. Each of us has all the genes that characterize the human species but with slight variations that convey genetic uniqueness to each of us. It’s these variations that determine an individual’s disease susceptibility and how s/he responds to particular environmental factors, such as food, medications, or environmental toxins. Defining the various factors involved and their interactions is expected to provide clinicians with the potential for more effectively managing existing disease and to be well positioned to prevent disease before it manifests.
Another important accomplishment of the Human Genome Project has been in the area of genetic technology. New technological advances have been developed, but even the original technologies have advanced to the point where analyzing the composition of the genetic material is considerably faster and cheaper than even a few years ago [1]. Ongoing development in genetic technologies is essential for genomics to achieve clinical utility. In order to predict a patient’s drug response or disease susceptibility, clinicians will need to know which gene variants are present within an individual’s genome. Significant progress is being made in developing fast, accurate, and inexpensive genetic testing and can be expected to continue so that test panels with clinical validity and utility will be readily available for widespread application in the near future. Expect testing to become increasingly simplified in administration but increasingly complex in clinical implications as knowledge expands and multiple gene–gene and gene-environmental factor interactions are discovered and associated with clinical outcomes. Systems biology and bioinformatics will be important behind-the-scenes fields supporting clinical application of the -omic technologies. Recent reviews address emerging technologies and disciplines and their importance to the development of genomics as clinically useful [2–4].
Oral health is of no exception when it comes to the important role of genes in function and is expected to benefit from the scientific foundation and resultant clinical applications of genomics [5–8]. Although the exploration of gene-based mechanisms in dental medicine is in its early stages, gene variants are being investigated for association with caries development, [9, 10] enamel formation, [11] and gingivitis and periodontitis [12–21]. A recent study in twins by Rintakoski and colleagues [22] includes a summary of the key research linking genes and oral disease. Among the clinical applications of genomics to oral health are pharmacogenomics and the prediction of patient response to medication; determining whether a patient is susceptible to being in a pro-inflammatory state, which promotes periodontal disease among other chronic diseases; predicting risk of caries formation; and nutritional genomics and its promise of matching diet and lifestyle choices to the underlying genotype of the individual in order to better manage existing chronic disease and prevent future disease.
Over the past decade, leaders in dental education have realized that genomics and molecular biology would need to be incorporated into dental practice and began to assess the preparation of dental professionals in these areas [23–26]. Behnke and Hassell [26] evaluated the genetics education requirements for admission into and graduation from dental schools and dental hygiene programs during 2003–2004. They found that none of the dental hygiene schools required a course in genetics for entry nor included one within the curriculum. Of the 54 dental schools, only one required a genetics course prior to entry and six had a formal, required course in genetics within the curriculum. In 2008, one of the three reports from the “New Models of Dental Education” study, funded by the Josiah Macy, Jr. Foundation, addressed genetics and its implications for clinical practice [5]. In this report, the panel considered the anticipated changes in dental practice and the knowledge, skills, and attitudes that would be required by dental professionals in an era of genetics and molecular biology. The report acknowledged the challenges of integrating these disciplines into the typically dense dental education curriculum and offered educational strategies for consideration. Despite the challenges, the fundamental role of genes in health and disease mandates the development of new knowledge and skills for dental professionals who can look forward to an expanded role in caring for the whole patient.
This chapter will provide the fundamentals of genomics, pharmacogenomics, and nutritional genomics and their anticipated applications to oral health and dental medicine.
Genomics
Because the information encoded within our genes is directly linked to our functional outcomes, we now realize that it’s important to understand gene-protein-function connections at the molecular level. This section provides a brief overview of the key aspects of how the genes direct the operation of an organism and how information from the environment communicates with the genes. This overview provides the background for understanding the utility of genomic applications, particularly pharmacogenomics and nutritional genomics, to dental medicine.
The Role of DNA
Deoxyriboncleic acid (DNA) encodes the genetic information of all living organisms. That such a chemically simple molecule can contain sufficient information for operating a living organism and that its expression is both carefully controlled and exquisitely responsive to environmental signals is a topic that has fascinated scientists for over 60 years. The molecule itself consists of two strands of nucleotide building blocks consisting of only three components: phosphorus, the 5-carbon sugar deoxyribose, and one of four nitrogen-containing bases: adenine (A), cytosine (C), guanine (G), or thymine (T). This foundational unit of DNA is called a “nucleotide.” To form each strand, nucleotides are covalently attached end-to-end in a linear arrangement, with the two strands being held together by complementary base pairing to form a double helix resembling a spiral staircase that winds upward in a right-handed direction. The sugar-phosphate moiety forms the railings of the staircase, with the bases on the inside forming the steps. The bases are held together by weak hydrogen bonds between complementary bases, with A pairing with T and G with C.
It is this linear sequence of bases that encodes the information that directs the synthesis of each of the proteins that does the work of the cells, thereby providing the organism with its functional abilities. This information is decoded in a complex but stepwise manner. It’s first transcribed from the DNA into messenger RNA (mRNA), a single-stranded molecule similar to DNA with ribose as the sugar and the base uracil (U) replacing thymine (T). DNA serves as a template for RNA polymerase to transcribe the DNA into mRNA. As with DNA, complementary base pairing directs the order of the bases of the growing mRNA strand, with the adenine (A) of DNA pairing with the uracil (U) of the mRNA. In humans and other higher organisms, the base sequence of DNA does not translate directly into the amino acid sequence of the protein and must first be processed to remove nucleotides not required for protein synthesis. Once processed, the mRNA is ready for translation into protein. Each set of three nucleotides in the information portion of the mRNA is a “codon” and corresponds to a particular amino acid, which during the translation process will be covalently connected in the correct order to yield the final protein structure. Proteins function in myriad ways: as enzymes, receptors, transporters, regulatory molecules, communicators and, in some instances, hormones. The protein’s function is directly related to its amino acid sequence, which determines its molecular folding and, thus, its activity. It’s easy to see the implications of an error in any step of this complex process. Introducing even a single alteration in the nucleotide sequence of DNA can potentially alter the amino acid sequence, the protein’s conformation and, thus, the function of the protein.
The total DNA contributes to the individual’s genotype; the outcome of the expression of the DNA into protein and function defines the phenotype. The genotype is essentially constant throughout one’s lifetime due to the stability of the DNA, but the phenotype can vary considerably since the expression of the DNA is subject to influence from a number of environmental factors.
Genes and Their Expression
A gene is a sequence of nucleotides in DNA that contains the information for synthesizing a protein. Data from the Human Genome Project suggest that humans have approximately 20,500 genes [27]. Genes have a characteristic architecture with three major regions: the regulatory region that controls how and when the encoded information is expressed, the promoter region to which the RNA polymerase attaches to begin transcribing the gene into mRNA, and the coding region that generates the amino acid sequence of the protein.
The regulatory region is of particular interest because of its ability to control gene expression and because it’s here that environmental factors communicate with the organism at the molecular level. Within the DNA sequence that forms the regulatory region of a gene are specific sequences that serve as “response elements.” Specialized proteins called “transcription factors” bind to the response elements and change the conformation of DNA, which allows or prevents RNA polymerase from binding to the promoter region and initiating transcription and, thus, gene expression. Transcription factors can have multiple binding domains. One domain binds to the response element but additional domains can bind small molecular weight ligands, such as nutrients, as well as other transcription factors. Depending upon the particular gene, a multimeric regulatory molecule may be required before the transcription factor complex is “active” and able to influence gene expression. In this way a gene’s expression is controlled in response to signals from the environment surrounding the cell. These signals may be transmitted by environmental toxins, xenobiotics (“new-to-nature molecules”), or naturally-occurring components within food, either conventional nutrients, phytonutrients, or other molecules in food that find their way intentionally or unintentionally into the food supply. Such molecules are called “bioactive food components” or, simply, “bioactives.”
The processes by which these environmental factors convey their message to the genetic material are complex in execution but elegantly simple in concept. In general, small lipophilic ligands can penetrate the cellular and nuclear membranes and interact directly with transcription factors that in turn bind to DNA and effect changes in gene expression. For example, omega-3 and omega-6 fatty acids serve as ligands for peroxisome proliferator activated receptors (PPAR), a transcription factor that regulates the expression of the information from a number of genes. Larger, less lipid-soluble ligands bind to cell surface receptors and elicit signal transduction, a cascade of reactions that results in one or more transcription factors binding to DNA and influencing gene expression. An example of this type of communication process occurs with the flavonoid class of phytochemicals, which binds to cell surface receptors, elicits signal transduction, and results in activation of various transcription factors such as nuclear factor kappa beta (NFkB) that in turn controls expression of a number of genes that contribute to the inflammatory and innate immune responses. Thus, by being able to sample the composition of the environment in which they exist and respond accordingly, organisms are able to adapt in beneficial ways that may increase their “goodness of fit” to their environment and improve their survival and reproductive potential.
Alterations to DNA: Changes in Chromosome Number or Structure
Although the focus of this chapter is on molecular genomics, the impact of changes to chromosome structure should be noted because such changes can ultimately impact DNA and its translation into the proteins needed for function. Chromosomes can be thought of as a packaging mechanism by which the large amount of DNA is packed within the microscopic nucleus. A chromosome represents a linear sequence of DNA nucleotides, with each gene and each nucleotide within that gene having a specific “address” on a particular chromosome. Should the normal number of copies of a chromosome or a chromosomal region be increased or decreased, the amount of information available is altered, which can result in more or less of a protein being produced. For the most part, alterations in the amount of genetic material are not well accommodated and these conditions typically lead to serious dysfunction/disease and often death. Similarly, breakage can occur in one or more chromosomes so that material is lost or its location changed (the “sticky” ends of broken chromosomes can append to another chromosome or the broken section can invert and insert “backwards” into the same location). When transcription of this rearranged DNA occurs, the information is often nonsensical, critical proteins are not made, and function is impaired. Such changes in chromosome number or structure are referred to as “chromosomal abnormalities” and will be seen in dental practice because individuals with these aberrations often have oral problems that can range from tooth morphological abnormalities to cleft palate to chewing and swallowing problems. The review by Jafarzadeh on taurodontism may be of interest in that it is often seen with Klinefelter syndrome (more than one X-chromosome in males), the most common chromosomal abnormality in humans [28, 29].
Alterations to DNA: Changes in DNA Sequence
There are two major classes of alterations to DNA at the molecular level, both of which can influence function: changes to the nucleotide sequence of DNA (called “genomic changes”) or chemical modification of either the proteins associated with DNA or the DNA molecule itself (called “epigenomics changes”). Epigenomic changes do not alter the nucleotide sequence of DNA and, thus, the information encoded within that sequence is not affected. Instead such changes affect gene expression. Epigenomics will be discussed shortly.
Changes to the DNA nucleotide sequence itself can occur at any point along the DNA. The functional impact of a change will depend upon which region of the gene is affected. In general, changes within the coding region impact the function of the protein and changes within the regulatory region impact the expression of the gene. Altering expression can impact the amount of protein produced but also affect the timing of production, which can have negative implications for normal development and for disease susceptibility. Thus, the functional impact of a change to the DNA sequence can be quite complex depending upon whether protein structure is affected, protein expression is affected (quantity and timing), and the nature of the environmental milieu bathing the genes.
When the change in nucleotide sequence occurs within the regulatory region, the impact is on the production of the protein rather than on its structure and function. Depending upon the specific nucleotide change, transcription may be increased (“up-regulated”) or decreased (“down-regulated”). This change may be negative, neutral or positive, depending upon whether transcription is increased or decreased. For example, it’s possible that a nucleotide change could affect the response element in the regulatory region or the transcription factor domain that binds to the response element, thereby changing the dynamics of gene expression. The altered binding may allow the gene to be constitutively expressed (always “on”), to be unable to bind appropriately and thereby unable to be expressed (always “off”), or any level of expression in between these two extremes, depending upon how strong the impact of the alteration is on expression. Changes to the ligand-binding sequence can alter the responses to environmental factors, increasing or decreasing gene expression. These nuances may seem minor but they have major impact when the outcome is whether or not a critical gene is expressed. For example, constitutive expression of the IL1 or IL6 genes, which are major determinants of susceptibility to chronic inflammation, could have far-reaching effects on an individual’s health, including predisposition to gingivitis and other periodontal diseases.
Further, a change in the regulatory sequence may occur in a gene that also contains a change in the coding sequence. In other words, it’s possible to have more than one change within the same gene (each nucleotide in the gene presents an opportunity for change).
Alterations to DNA: Changes in the Epigenome
The second type of alteration to DNA is the basis for the developing field of epigenetics/epigenomics, which here will be referred to for simplicity as “epigenomics.” First consider that there are approximately 3 billion DNA nucleotides in the human genome that are housed within the microscopic nucleus. A packaging mechanism is needed, similar in concept to the need to condense computer data in order to fit large amounts onto a hard drive. Chromosomes serve as the packaging mechanism for higher organisms. Humans have 23 pairs of distinct chromosomes, one member of each pair contributed by each parent. Important components of the condensation mechanism are histones, positively charged proteins that assemble into sets of eight units called “nucleosomes.” Similar to winding thread around a spool, DNA winds around each of the nucleosomes and a highly condensed structure called “chromatin” results, the complex of DNA-histone.
When DNA is condensed, it is not available for transcription. In order to be physically accessible to enzymes that can duplicate or transcribe the DNA, chromatin must be relaxed. Condensation and relaxation are controlled through covalent attachment of chemical groups, such as acetyl groups, to the histone proteins. In the absence of acetylation, chromatin is condensed. Addition of acetyl groups through acetylation relaxes the DNA so that it can be transcribed and the information in the genes expressed. Deacetylation closes the chromatin and impedes transcription.
There is an additional level of modification that further regulates DNA expression and that is the covalent attachment of specific chemical groups, such as methyl groups, directly to the DNA. In general, methylation of DNA silences its expression; demethylation permits expression. This system is superimposed onto the DNA sequence to provide an additional level of control of gene expression and has been likened to Nature’s pen-and-pencil set for its ability to be modified by diet and other environmental influences [30].
Further, epigenetic markings (such as the methyl profile or “signature” of DNA) can be inherited and are influenced by the markings of ancestors at least two generations removed from a current generation. In other words, the diet and lifestyle choices of our grandparents influence our functional abilities [31, 32]. Predictably, nutrition will factor prominently into our understanding of the connection between our genes and our functional abilities and will be a critical determinant of our being able to reach our full genetic potential.
More questions than answers exist concerning epigenomics and its impact on human health. We do know that epigenomics is of major importance in development and differentiation because the turning on and off of genes and the timing of this activity is critical for these processes. Alterations in the developmental program can have profound effects on a fetus. Although it is possible that an alteration may provide an evolutionary advantage to an organism, more examples of detrimental effects are known at this time than beneficial ones. Similarly, in carcinogenesis there are genes (oncogenes) that, when expressed, promote uncontrolled growth and other genes (tumor suppressor genes) that keep such growth under control. Inappropriate methylation of either type of gene could lead to carcinogenesis. For additional information about epigenomics, see recent overviews by Hirst and Marra [33], Villagra et al. [34], Rakyan et al. [35], Park et al. [36], Romagnolo et al. [37] and Stein [38].
Genetic Alterations and Function at the Molecular Level
Clearly changes in DNA or in its epigenomic “markings” are important to our ultimate functioning and to our health and disease susceptibilities. The terminology for such changes is in transition. In this section, the spectrum of biological outcomes resulting from changes in DNA sequence or epigenomics markings is explored. An attempt is made to convey understanding within the present context and to point out limitations to current terminology and how it’s likely to change as both knowledge and technology expand. A number of familiar disease states are used to illustrate how understanding of “genetic disease” is undergoing major revision as the molecular basis for disease is now being explored. The important point is that the extent to which function is influenced by changes to genes can that vary from severe to mild depending on the role of the gene and where within the gene the change occurs, which may affect expression of the gene or the structure of the protein if the gene is expressed. Further, for many genes, a change may have no effect on function unless the gene interacts with one or more environmental factors. This latter mechanism seems to occur commonly and to be associated with chronic diseases.
Historically, linking a change in DNA to a change in protein expression or function and ultimately to a disease was a major breakthrough in understanding the critical role of genes in health and disease. Such diseases were originally called “genetic diseases” and thought to be a distinct type of disease that occurred rarely but was typically severe in its impact, which manifested early in life. When such changes were in genes and proteins involved in metabolic reactions, the disease was called an “inborn error of metabolism.”
Traditionally the term “mutation” was used to describe a rare change in the DNA sequence, which typically resulted in a detectable disease condition. However, as technology improved, more subtle subclinical effects were detected. In addition to the severe forms of disease, milder forms could be traced to a change in the same gene and, ultimately, technological advances allowed detection of changes that led to increased susceptibility but not necessarily to overt disease. These types of mutations came to be called genetic “variations” and the genetic basis ascribed to “gene variants” in which the DNA nucleotide sequence had been altered. In many cases only when the variation interacted with specific environmental factors did disease arise. The term “single nucleotide polymorphism” (SNP, pronounced “snip”) referred to those variations in which a single nucleotide change occurred and the variation was frequent in the population (initially defined as occurring in >1% of a population). More recently, alterations that change the epigenetic signature of the DNA have expanded the lexicon. The lines between the mutations and genetic variations have begun to blur as technological advances have enabled the discovery that many diseases have a spectrum of symptoms, ranging from severe to mild, regardless of the specific type of change involved or whether it occurs rarely or frequently within a population. The key defining characteristic appears to be the impact of a genetic alteration on functional outcomes.
Cystic fibrosis, sickle cell disease, and the inborn error of metabolism phenylketonuria are classic examples of rare single gene disorders that cause disease and are considered to result from “mutations.” Historically, when cystic fibrosis was diagnosed, genetic analysis confirmed the presence of two copies of a mutation in the cystic fibrosis transmembrane conductance receptor gene (CFTR). Over 1900 mutations have now been identified in this one gene, some of which lead to severe symptoms and others to a mild condition [39]. The changes have occurred in the same gene in all cases, but the nucleotide involved and the impact on function is the determining factor as to the severity of the outcome. If one of these changes in the CFTR gene is found to be common within a population, it might now qualify as a “gene variant” rather than a mutation.
Sickle cell disease also offers a dramatic example of the effect of a change in a single nucleotide on function and health. The substitution of a T for an A in the HBB gene sequence that encodes the beta-globin subunit of hemoglobin leads to the neutrally charged amino acid valine replacing the negatively charged glutamic acid. The resultant conformational change to the peptide decreases its ability to bind oxygen. Those with two copies of this alteration exhibit a sickled shape to their red blood cells and the painful symptoms of sickle cell disease under conditions of low oxygen tension. Further, those with a single copy have an intermediate phenotype, sickle cell trait. Not only does the actual change and its impact on function define the phenotype but the number of copies of the change (gene dosage effect) is also an important determinant of the biological outcome. Molecular and clinical details of this disorder can be found in reviews by Frenette and Atweh [40] and, more recently, by Steinberg and Sebastiani [41].
The inborn errors of metabolism (IEM) in which the impact is nutritionally related are similar in concept to cystic fibrosis and sickle cell disease in that they occur rarely but yield a detrimental functional impact. The classic IEM example is phenylketonuria (PKU) in which a single nucleotide change within the PAH gene leads to the absence of phenylalanine hydroxylase. This change prevents the conversion of the amino acid phenylalanine to tyrosine and creates a life-threatening deficiency in tyrosine if it is not supplied in the diet. It also leads to an accumulation of phenylalanine and its metabolites, such as phenylpyruvate, which can be detrimental to the developing brain. An overview of PAH and its relationship to PKU from an historical and contemporary status is provided by Zurfluh et al. [42].
As knowledge of molecular nutrition and molecular genetics increases, multiple phenotypes for PKU and other IEM have been detected. The review by Gersting et al. [43] of known PAH mutations suggests that a variety of types of changes is represented, all impacting the ability to ultimately generate tyrosine from phenylalanine. The spectrum for phenylalanine tolerance varies from PKU, which requires a phenylalanine-restricted diet, to mild hyperphenylalaninemia that does not require dietary restriction. This phenotypic spectrum appears to mirror the impact of the various changes on function and is emerging as a common theme as knowledge of the molecular basis for disease expands.
An example of a mutation that would clearly be labeled a “gene variant” is the 677C > T change in the MTHFR gene. This gene codes for the enzyme methylenetetrahydrofolate reductase that converts folate into its 5-methytetrahydrolfolate active form, which in turn is involved in the conversion of homocysteine to methionine as well as supplying other metabolic reactions with methyl groups [44]. Insufficient 5-methyltetrahydrofolate can lead to elevated levels of homocysteine, which have been linked to increased risk of cardiovascular disease [45]. A cytosine-to-thymine change at nucleotide 677 of the DNA leads to an enzyme that is impaired in its ability to supply adequate levels of active folate. With this particular nucleotide change, the impairment is not sufficient in itself to cause dysfunction and a disease state typically does not manifest unless the individual consumes insufficient folate. Dietary folate insufficiency, however, is suspected by the World Health Organization of being a common global problem [46].
Virtually all of the chronic diseases that plaque modern society, such as heart disease, cancers, diabetes, inflammatory disorders, osteoporosis, and obesity, are caused by changes that can be classified as genetic variations. A change in a single nucleotide may occur along with other single nucleotide changes that alone are minor in functional impact but increase risk in the aggregate or may interact with one or more environmental factors to promote dysfunction and disease. Additionally, changes impact the epigenetic markings of DNA and chromatin and interfere with access to the expression of one or multiple genes. Most likely these changes will also be found to fall at both ends of the mutation versus variation spectrum and points in between.
It should be apparent that the clear distinction between mutation and variation is becoming murkier, not clearer, as knowledge expands. Even the classic disorders such as sickle cell and the IEM are now known to have a spectrum of phenotypes that can be correlated with particular genetic changes and their impact on function. Clarity will not likely be achieved until large numbers of genetic alterations have been studied within multiple genes and the environmental triggers have been identified. The key points here, though, are that a single nucleotide change can have devastating consequences to function or may be silent unless interaction with an environmental trigger occurs. Further, genetic disease appears not to be a type of disease but underlies essentially all disease, with the severity reflecting the impact of the genetic alteration on function. Similarly, the earlier thinking that classified people as “normal” or “abnormal” with respect to a disease is giving way to an understanding that each person is genetically unique. Ultimately clinicians will need to integrate this new shift in thinking into every aspect of patient care, which includes dental medicine. Genomics will be incorporated into diagnosing oral disease, predicting susceptibility to developing cavities, defining a patient’s drug-metabolizing capacity, and determining dietary requirements.
Detecting Alterations in the Genome
Genomics and its related -omic disciplines have the potential for fostering the development of personalized healthcare, in which the genetic makeup of each individual can guide health-related decision making. In order to realize this potential, there must be a way to analyze the DNA sequence and its epigenetic markings. The basic technology for genetic testing has been in use for decades and new refinements and enhancements are continually being added. What lags at this point is the research foundation for associating phenotype with genotype. That is, we are in the early stages of associating defined gene changes (mutations or variations or epigenetic markings) with specific disease states and with particular gene-environmental factor interactions that can convert susceptibility into disease or offer effective intervention approaches. The following section provides an overview of the ongoing importance of using family history and genetic analysis to detect disease susceptibility.
Family History
Family history has long been used to predict the potential disease susceptibility of patients by detecting patterns of inherited disease within their extended family. Although there are a number of limitations to family history as a predictor of disease, this method will continue to be of primary importance until the point at which it becomes economically feasible and clinically relevant to assess the genome of humans directly.
In addition to it being time-consuming to gather genetic history information and many clinicians are not trained in producing and analyzing the resultant pedigrees, there are other important limitations. Family history is most useful for those diseases that result from genes that are inherited by a recognizable mode of inheritance (Mendelian or mitochondrial) and have a sufficiently strong impact on function that when present they are detectable in the phenotype. Additionally, family history is only as accurate as the information that goes into it. Many individuals do not know their family history; many families do not openly discuss topics that are relevant to family history, such as abortions, miscarriages, incest, marriage among close relatives, and family members with mental health conditions. Others are adopted and their biological history may not be known. To the extent possible, the clinician should strive to obtain a family history that covers at least three generations. Numerous forms for collecting family history exist. The U.S. Surgeon General has recently initiated a nationwide drive to help people become more aware of their family history and to document the information to be shared with their various healthcare providers (www.hhs.gov/familyhistory). Family history provides a useful service in raising awareness of inherited disorders that patients may not realize exist within their family but is limited by its inability to detect many of the chronic disorders that providers routinely encounter today.
Genetic Testing
Genetic testing is increasingly being used in healthcare settings. The type of testing may be chromosomal analysis to detect changes in number or structure of the chromosomes or DNA analysis for detecting particular mutations, SNPs, epigenetic modifications, or changes in the number of copies of a gene.
Considerable research attention is being directed toward identifying SNPs associated with a variety of chronic diseases and developing genetic tests. Several tests are presently available for use by providers and consumers. This application of genetic testing is in its infancy but holds considerable promise. The technology used for this type of testing is sound as it is the same as that used for decades in research and clinical labs to test for inborn errors of metabolism and other single gene, rare disorders. The limitation at present is the paucity of research validating the associations between particular SNPs and susceptibility to disease and the associations between particular interventions and improved disease management or prevention. However, genetic testing is expected to be an increasingly fruitful approach as research continues to move forward, providing a much-needed mechanism for identifying disease susceptibility that often cannot be determined through family history. Further, through genetic testing, disease susceptibility can be detected prenatally and can be used to guide diet and lifestyle decisions early in life, with the goal of preventing disease from developing.
Genomic-Related Applications to Oral Health and Disease
The fundamental relationship among genes, gene alterations, protein, and biological function at all levels predicts that gene-related principles will become increasingly integrated into healthcare, including oral healthcare [23–26]. A heightened awareness of the value of including the broad scope of genomics in the dental school curriculum has been apparent during the past decade [5, 26]. This section provides examples of how genomics, pharmacogenomics, and nutritional genomics are presently being applied clinically.
Genomics
Genetic analysis has long been used clinically to confirm a diagnosis. Once the gene-disease association has been established and the more common mutations identified, it became possible to confirm that an individual has a particular genetic change within a gene known to be responsible for the disease. Clinical applications of genomics also include distinguishing among similar tumor pathologies to arrive at an accurate diagnosis, determining the molecular nature of tumors for diagnostic and treatment purposes, monitoring prognosis and early treatment failure, and identifying microorganisms and viruses that may be causative agents in disease.
A particularly active research area has been the application of genomics to various aspects of cancer therapy. Notable successes include tailoring treatment to the genetic characteristics of the cancer or to the individual’s genotype as well as detecting early treatment failure [47]. For example, genomic information has been critical in successful outcomes achieved with the topoisomerase-1 inhibitor irinotecan (Camptosar®) for metastatic colorectal cancer, [48, 49] the tyrosine kinase inhibitor imatinib (Gleevec®) for chronic myelogenous leukemia, [50, 51] and trastuzumab (Herceptin®) for breast cancer [52].
Using genomics to predict future disease is expected to be a major application, with sample collection being noninvasive and as simple as a buccal swab or saliva sampling [53, 54]. Detecting potential susceptibility to disease can help the patient prevent disease as well as alert family members who are at risk and educate them as to their options for disease prevention. Once the specific mutation is known, family members can be tested to see whether they carry that same mutation. This procedure has been used for several decades for the inborn errors of metabolism, cystic fibrosis, sickle cell disease, and numerous other well-characterized single gene disorders. The limitation of this approach is that a negative result can be equivocal. If an individual tests negative for known mutations, it is still possible that the individual has a mutation in that gene but that the specific mutation has not yet been identified (and, thus, would not be detected by current genetic tests).
This scenario is, unfortunately, common at present because genetic technology primarily identifies the more common mutations and tests for their presence. Two enhancements are expected to solve this limitation in the near future. One is the ongoing identification of new mutations by researchers. Another is the increasing use of DNA sequencing to detect genetic changes. In DNA sequencing, the nucleotides within the gene are identified as to composition and order and compared against a DNA sequence that research has shown to be the gene sequence that commonly occurs in healthy individuals. Ultimately the entire genome will be routinely sequenced and analyzed against sequences typical of healthy people in order to detect where an individual differs from the healthy state. The methylation status of the DNA is an additional important characteristic and can also be detected using current technology.
Recent reports of genomic approaches related to dental medicine include a diverse set of applications. Analysis of the amelogenin genes is a useful laboratory procedure in archeology, forensic dentistry, and research [55–57]. The analysis can be used for sex identification as well as for quality control purposes to ensure that sample mix-up has not occurred. DNA sequencing is used to confirm the occurrence of particular diseases within a population, such as the recent identification of Shwachman-Diamond syndrome in a population in which this disorder was not thought to occur [58].
Clinical applications to dental medicine are equally diverse and are best characterized as being in the early stages of development. Detection of genetic alterations can be used for a wide spectrum of applications, from distinguishing pathologically similar disorders to predicting susceptibility to various oral diseases, such as caries development, [59] periodontal disease, [60–63] and oral cancers [64, 65]. As has been noted for oncology in general, genetic analysis is being applied to oral oncologic disorders for accurate diagnosis, treatment selection, and monitoring treatment failure. For example, in the pathologically similar epithelial odontogenic tumors such as ameloblastomas and clear cell odontogenic carcinomas, DNA analysis is used to aid in accurate diagnosis [66].
Genomics can also be helpful for identifying the underlying molecular basis for noncancerous disorders. Amelogenesis imperfecta (AI) is a group of disorders that involve changes to at least 5 genes involved in enamel formation that give rise to at least 14 subtypes [67–69]. Genomics is helpful in accurately indentifying the underlying molecular basis for AI as well as in predicting the risk to family members [70–79]. The various types of AI are inherited in an autosomal dominant, autosomal recessive, or X-linked recessive manner. Since these traits have different modes of inheritance, they have different predictions of susceptibility for family members, depending on the gene involved and its mode of transmission. In a recent publication, Lindemeyer and colleagues predict that classification of AI will shift from pathology-based to gene-based before long [80].
Amelogenesis imperfecta is but one of many illustrations of how SNPs will be used to predict increased risk of disease. A major application is expected to be in detecting susceptibility to caries development in children so that early preventive treatment approaches can be initiated [5, 81]. Another example applicable to dental medicine is the finding that a combination of SNPs within the alcohol dehydrogenase gene (ADH) and the methylenetetrahydrofolate reductase gene (MTHFR) is associated with increased susceptibility to oral squamous cell carcinoma when the susceptible individual consumes alcohol [82]. Early detection of this susceptibility affords the individual the choice of taking steps that could prevent the disease from manifesting.
The gene for each protein involved in the various oral diseases provides the potential for susceptibility testing once the gene-disease associations have been adequately explored. As an increasing number of gene-oral disease associations are identified and their molecular mechanisms determined, the clinical utility of predictive genomics is expected to expand considerably.
Pharmacogenomics
Pharmacogenomics focuses on the genetic basis for the patient variability in response to drug treatment. Dental practitioners may prescribe medications (1) to control pain or anxiety, (2) as part of the treatment of specific oral conditions, or (3) to eradicate or prevent infection. The agents used may include nonprescription and prescription anti-inflammatory agents, narcotics as well as various milder analgesics, antibiotics and other antimicrobials, and muscle relaxants. These agents are typically metabolized in the gastrointestinal tract and the liver in a two-phase process referred to as Phase I and Phase II biotransformation. In many cases drugs require biotransformation for biological activity, but the endproduct of this process is the generation of a metabolite that can be more readily excreted (“cleared”) from the body than the original agent.
Phase I biotransformation involves the cytochrome P450 (CYP 450) family of oxidoreductases that generate a highly reactive intermediate. The majority of drugs marketed today are metabolized by the cytochrome P450 enzymes [83, 84]. The intermediate is subsequently conjugated by Phase II enzymes, either with a glutathione moiety (via the family of glutathione-S-transferases, GSTs), an N-acetyl group (via the N-acetyltransferase enzymes, NATs), or a glucuronic acid moiety (via the UDP-glucuronosyltransferases, UGTs). Sissung et al. [85] and Pacheu-Grau and colleagues [86] provide overviews of the pharmacogenomics of drug-metabolizing enzymes in general, and Hersh and Moore [87] address adverse reactions in medications used in dentistry.
As with any gene, those that encode the major drug-metabolizing enzymes can acquire changes that can affect the activity of these enzymes and, thereby, patient response to medications. Each patient’s set of variants within the genes that encode the major drug-metabolizing enzymes provide a personalized profile in terms of response to medications. The response can vary from beneficial to neutral to harmful, depending upon the drug and the person’s gene variants. Alterations in the Phase I or Phase II genes can influence the enzymatic conversions and either enhance toxicity or decrease it. For example, lidocaine, erythromycin, and the benzodiazepines are metabolized primarily by cytochrome P450 3A4. Impaired enzymatic activity will result in unmetabolized drug. Not only is this individual not receiving the drug effect intended, the unmetabolized molecule can accumulate and lead to toxicity. If Phase I activity were normal but Phase II impaired, the drug would still not be fully converted to its active metabolite so tissue levels would not reach the required concentration of drug. Additionally, the slow Phase II reaction causes accumulation of the reactive intermediate that, as a reactive oxygen species (free radical), is potentially harmful in itself. Fortunately, nutrition is an important component in the optimal functioning of Phase I and II enzymes from a number of standpoints and can be used to improve these reactions, as will be discussed in the “Nutritional Genomics” section.
There are other enzymes of importance beyond the Phase I and Phase II systems, such as the catechol-O-methyltransferase encoded by the COMT gene and the enzymes that provide defense against free radicals, such as the superoxide dismutases (SOD). The three main SOD enzymes—SOD1, SOD2, and SOD3—are slightly different in that they are coded for by distinct genes, differ in their coactivator molecules, and are located in different cellular compartments or in the extracellular matrix. The COMT enzyme is important in estrogen metabolism but is also involved in an individual’s sensitivity to pain [88].
Although it is common to speak of adverse drug events as though the drug were the problem, the underlying basis for a patient’s response is not necessarily something adverse about the drug but almost certainly an inappropriate match between the drug and the patient’s genes that encode the drug-metabolizing enzymes. At a population level, there is typically a spectrum of responses to a particular drug at a specific dose. Often the response for an individual in that population is reproducible for a given drug taken at a given dosage because the response reflects that person’s gene-directed ability to metabolize the drug. In the future it is likely that dental professionals will have as part of the dental record the genetic profile of a patient’s drug-metabolizing enzymes, which can be helpful in selecting appropriate medications.
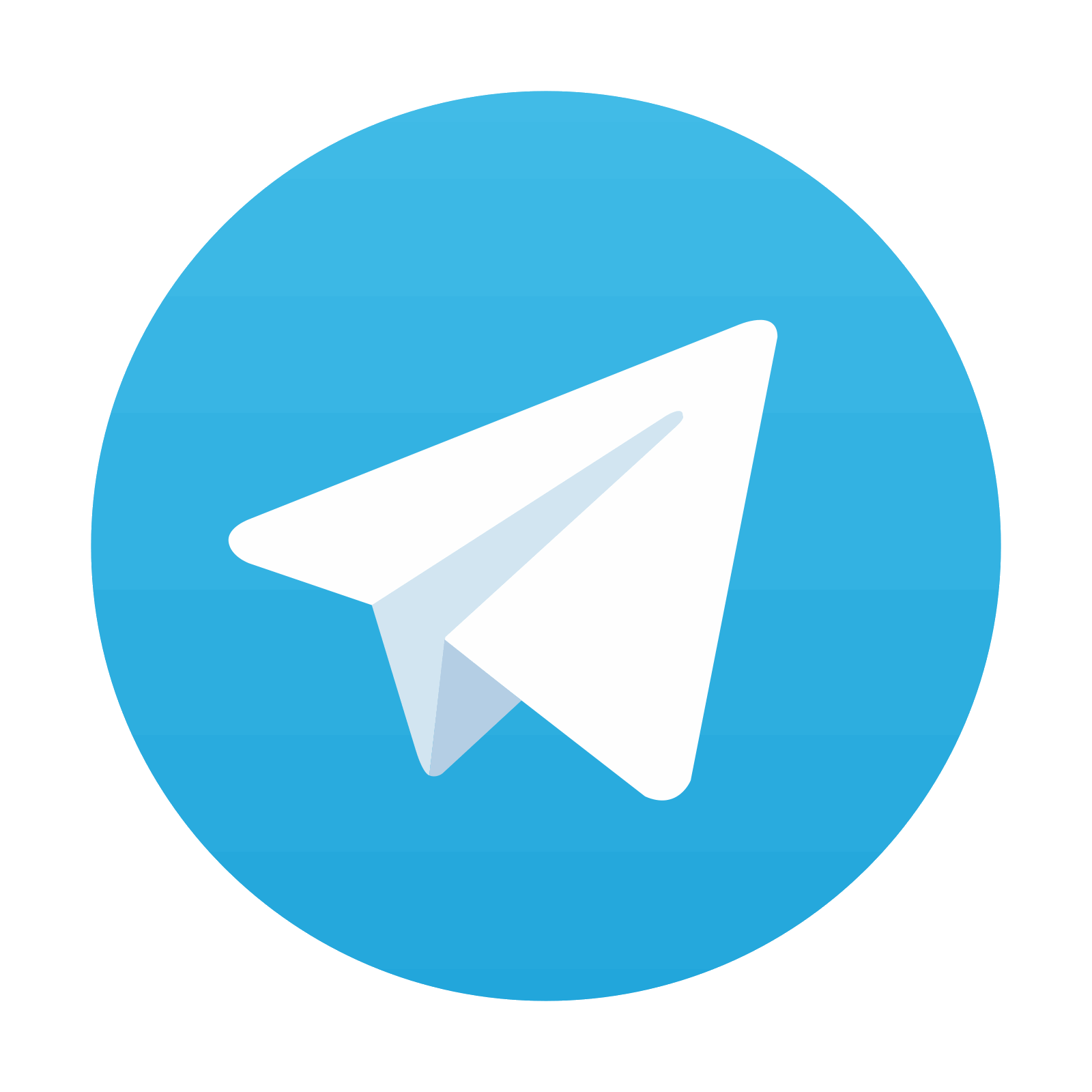
Stay updated, free dental videos. Join our Telegram channel

VIDEdental - Online dental courses
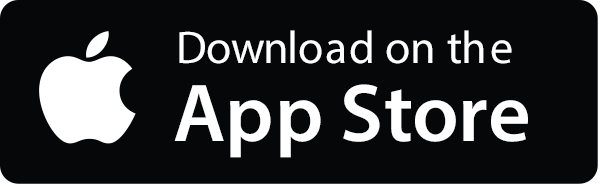
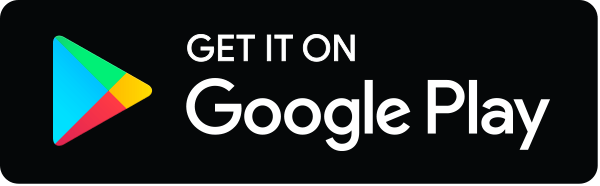