, Hai-Yang Yu2, Jing Zheng1, Lin-Mao Qian1 and Yu Yan3
(1)
Tribology Research Institute, Southwest Jiaotong University, Chengdu, People’s Republic of China
(2)
West China College of Stomatology, Sichuan University, Chengdu, People’s Republic of China
(3)
Institute of Advanced Materials and Technology, University of Science and Technology, Beijing, People’s Republic of China
Abstract
This chapter deals with the friction and wear behavior of human teeth. Human teeth are the important masticatory organ in body. In general, oral biomechanical functions can result in tribological movement of teeth occurring in the mouth [1–4]. Hence, tooth wear is a cumulative multifactorial lifetime process, which to a large extent is irreversible [5, 6]. However, excessive wear may lead to a lack of perfect occlusal contact (the contact related to occlusions of the teeth, especially on the chewing or biting surfaces), a lower mastication efficiency, and an obliteration of the chewing surface [7–12]. Understanding the friction and wear behavior of human teeth would help deepen the scientific knowledge about human teeth and then provide valuable insights into the development of advanced dental materials, oral treatments, as well as the biomimetic design of an antiwear engineering system based on human teeth.
3.1 Introduction
This chapter deals with the friction and wear behavior of human teeth. Human teeth are the important masticatory organ in body. In general, oral biomechanical functions can result in tribological movement of teeth occurring in the mouth [1–4]. Hence, tooth wear is a cumulative multifactorial lifetime process, which to a large extent is irreversible [5, 6]. However, excessive wear may lead to a lack of perfect occlusal contact (the contact related to occlusions of the teeth, especially on the chewing or biting surfaces), a lower mastication efficiency, and an obliteration of the chewing surface [7–12]. Understanding the friction and wear behavior of human teeth would help deepen the scientific knowledge about human teeth and then provide valuable insights into the development of advanced dental materials, oral treatments, as well as the biomimetic design of an antiwear engineering system based on human teeth.
Human teeth possess a unique structure composed of enamel, dentin-enamel junction (DEJ), dentin, and pulp; each zone is anisotropic due to the enamel rods’ orientation, dentinal tubule, etc. [13]. Enamel is the hardest tissue in the human body because of the existence of enamel rods and is a composite consisting of both a mineral phase and an organic phase. Compared with enamel, dentin is widely considered to be elastic and soft. The study of Xu et al. indicated that microcracks formed on enamel interact with the DEJ and the enamel rods strongly and that the mechanical properties of teeth are functions of microstructural orientations [14]. The mechanical properties, chemistry, and microstructure of enamel in a maxillary second molar (M2) have been characterized as a function of location on an axial cross section [15]. Based on the previous results, it is reasonable to deduce that the tribological behavior of human teeth also interacts strongly with the microstructure’s orientation and location.
Wear of human teeth is an extremely complex process that involves mechanical, thermal, and chemical reactions. The wear rate of teeth may be closely associated with human physiological factors (such as age, gender, etc.) and pathological factors [7, 8, 16, 17]. For young people, enamel of a 2–3-mm thickness serves as an occlusal surface. With aging and various pathological factors, the enamel will be gradually ground down by mastication. As a result, superficial dentin will be exposed and worn away partially. Compared with enamel, dentin has more organic substances, and thus a lower hardness and a lower wear resistance. Clinical studies showed that the exposure of dentin on the occlusal surface resulted in a high sensitivity of teeth to normal irritations, a low mastication function, and so on [7, 18]. To some extent, the longer the service lifetime of enamel in the mouth is, the healthier human teeth are. Therefore, studying the detailed wear behavior of human tooth enamel is imperative to reveal the wear mechanism of enamel, to help clinical treatment for teeth, and to develop new dental restorative materials.
As mentioned in Chap. 2, the marked advantage of in vitro testing is to analyze fundamental mechanisms, which may lead to a better understanding of in vivo failure patterns. Many in vitro methods have been developed since the 1990s. Comparing the results obtained by different devices was difficult. In this chapter, we investigate the friction and wear behavior of human teeth mainly based on our own in vitro research results. Some progress in these topics from the literature is also reviewed. The next section will proceed from the friction and wear behavior of human teeth at different locations and deal with the wear–microstructure interactions of human teeth. The third section covers the wear process and mechanism of human tooth enamel. The fourth section discusses the effect of age on the tribological behavior of human teeth. Furthermore, the effects of pathological factors and toothbrushing are reviewed in the fifth and sixth sections, respectively.
3.2 Effect of Tooth Microstructure on Its Friction and Wear Behavior
In this section, detailed research is outlined on the friction and wear behavior of human tooth enamel and dentin. The wear–microstructure interactions were examined by evaluating wear behavior in both the axial and occlusal orientations and in different positions [19]. Particular attention was paid to the effects of enamel, enamel rod orientation, and dentin on the tribological behavior of teeth.
Flat samples used in this study were freshly extracted human teeth without caries. The teeth were placed in distilled water at 4 °C to avoid dehydration before sample preparation. Before testing, each tooth was cut into two halves using a diamond saw, with the cut lying perpendicular to the buccolingual division line. The cutting was conducted under water cooling, aiming at mitigating local overheat, which can result in dehydration and changes in the microstructure and chemistry of human teeth. Each tooth half was embedded into a steel-made mold with self-setting plastic (10 × 10 × 20 mm in size). One half of each tooth was ground and polished down to 0.5 mm on the occlusal surface (this orientation is referred to as the “occlusal section”), while the other half of each tooth was ground and polished on a plane perpendicular to the occlusal surface, removing approximately one fourth of the tooth, so that the polished surface intersected the cusp tips and exhibited the maximized enamel thickness (this orientation is referred to as the “axial section”). Samples were first ground using abrasive papers and then followed by diamond paste polishing. After polishing, the samples were stored in distilled water at 4 °C. The teeth were dehydrated partially during preparation, but efforts were made both to shorten the dry time and to keep the preparation time approximately the same for each sample.
The microhardness of each tooth contact surface was tested before the wear test. Ten to twenty indentations under a 50-g load were produced for each polished surface. In vitro wear tests were conducted in a ball-on-flat configuration using a reciprocating apparatus containing an artificial saliva solution; see Fig. 3.1. Pure titanium has often been used clinically as a dental material recently due to its excellent biocompatibility. Thus, high-purity titanium (C 0.10 %, Si 0.15 %, H 0.02 %, O 0.20 %, N 0.05 %, Fe 0.30 %, Ti the balance) with a hardness of 240 HV50g and 40 mm in diameter was used as a ball counterpart. For the occlusal section of each tooth, wear test was first performed on sample surface. After microscopic examinations on the morphology of the wear scar and measurements of wear depth, the worn surface was removed by about 0.5 mm through the grinding and polishing process. A new surface was obtained as a result, ready for a second wear test. According to this method, wear tests were performed gradually from the outer enamel to the dentin in every sample. For the axial section, wear tests were conducted in the enamel, DEJ, and dentin, respectively, with the three wear scars aligned.
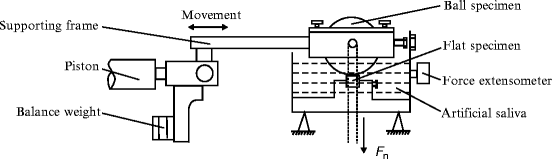
Fig. 3.1
Schematic diagram of friction and wear-testing rig [19]
The artificial saliva (its composition is listed in Table 3.1) was prepared according to Fusayama’s guidelines with Holland’s modification [20, 21]. In order to avoid the effect of acidity on wear, artificial saliva was neutralized to a pH of 7. A normal force of 20 N, a reciprocating amplitude of 500 μm, and a frequency of 2 Hz were used for all the wear tests. To better simulate the real wear conditions of human teeth, the choice of these parameters is based on clinical experience and the literature. During humans’ normal chewing process, the magnitude of the masticatory force in the oral cavity ranges from 3–36 N [22]. Also reportedly, the loads applied to the teeth during normal chewing are in the range of 10–20 N at the initial contact [23, 24] and are increased within the range of 50–150 N at the end of the chewing cycle [25, 26]. The magnitude of the force depends mainly upon the physical properties of food. As there are a great variety of foods, various forces can be expected. In addition, the maximum biting forces vary according to gender, age, and muscle build. Patients with bruxing habits can apply occlusal loads of approximately 1,000 N [27]. This work focused on the friction and wear behavior of human teeth during normal chewing. Under the applied normal load of 20 N, according to Hertzian contact theory [28], the associated mean contact pressure (208.0 MPa) was below the yield strength of enamel (330 MPa) [14, 29].
Table 3.1
Composition of artificial saliva
NaCl
|
KCl
|
CaCl2.2H2O
|
NaH2PO4.2H2O
|
Na2S. 9H2O
|
Urea
|
Distilled water
|
---|---|---|---|---|---|---|
0.4 g
|
0.4 g
|
0.795 g
|
0.78 g
|
0.005 g
|
1 g
|
1,000 ml
|
In the occlusal section, from the enamel to the dentin (Fig. 3.2a), friction logs describing the variation in the coefficient of friction, as a function of the number of cycles, are shown in Fig. 3.2b. Near the occlusal surface, an enamel layer of about 2 mm on the tooth crown showed excellent friction behavior. A lower friction coefficient was observed at the early stage. The coefficient was about 0.10 and remained constant up to 1,600 cycles (Fig. 3.2b). Then the coefficient increased rapidly to 0.80 and finally remained steady at about 0.85. With the enamel layer being thinned by mechanical polishing, the evolution of the coefficient was found to be similar to each other, while the number of cycles of the low friction coefficient, prior to the transition to a higher value, was reduced (Fig. 3.2b). Additionally, the evolution of the friction coefficient near the DEJ zone exhibited that the lower coefficient only lasted for about 50–100 cycles, and then the coefficient increased. When friction occurred on the superficial dentin, the coefficient increased rapidly from the first cycle. The observations suggest that the friction behavior changes from location to location for the same tooth.
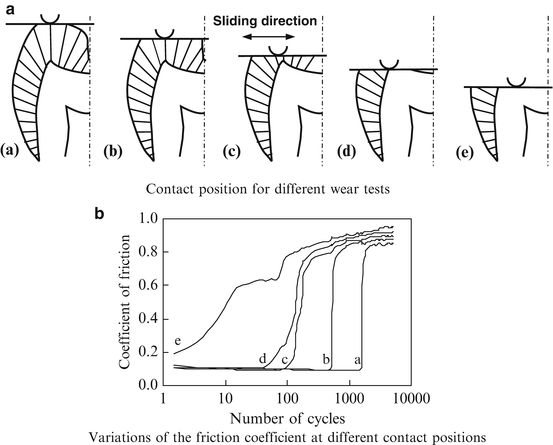
Fig. 3.2
Friction behavior of the natural tooth parallel to the occlusal section [19]
Laser scanning microscopy examinations after wear tests show three typical wear scars from the enamel to the dentin in the occlusal section, as shown in Fig. 3.3. Many particles were attached to the worn surface of enamel (Fig. 3.3a), while a strong plough effect (Fig. 3.3b) due to abrasive wear along the motion direction was observed on the worn surface of dentin, with many dentinal tubules (black dots in Fig. 3.3b). In the DEJ, two different regions appeared clearly on the contact surface (Fig. 3.3c). The region on the left side was covered by many particles, and the region on the right side was full of ploughs. Obviously, a significant difference existed in the morphology of the wear scar between the enamel and the dentin. Based on the fact that enamel is more fragile than dentin, one could infer that the particles obtained at the worn enamel are mainly the products of microcracking induced in the process of wear, and the ploughs are consequences of plastic deformation. The inference is also supported by the result of Xu et al. [14], who found that during the indentation of human teeth, cracks were observed in enamel with a low indentation energy, while no cracks were observed for dentin with a high indentation energy. This behavior was explained by a different cutting mechanism [14]. That is, the enamel was removed by a microfracture process, whereas the dentin was probably removed by a ductile chip formation process.
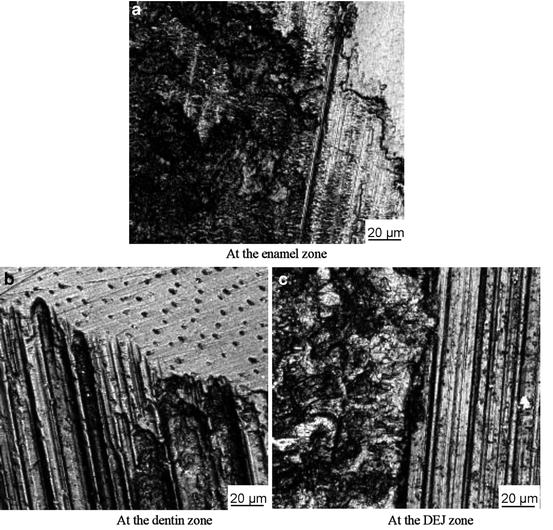
Fig. 3.3
Three types of wear scars observed parallel to the occlusal section [19]
A profile measurement shows significant increases both in the depth and in the area of the wear mark from the enamel to the dentin, as shown in Fig. 3.4. But the results of the layer-to-layer variation in the hardness (H) exhibit an opposite trend: H decreased by 17 % from the outer layer of enamel to the DEJ and further by 77 % from the DEJ to the dentin (Fig. 3.5). Each value of H was the average of 10–20 indentations under the same load in one layer. The standard deviation for these average values ranged from 2HV, for the dentin, to 20HV, for the vicinity of the DEJ. It implies that the H-value is different not only between the enamel and dentin, but also between different occlusal layers of the enamel for a tooth. Cuy et al. also suggested that such mechanical properties as H and the elastic modulus (E) of human enamel in a maxillary M2 were the function of position in an axial cross section [15]. The difference in H of different layers of a tooth clearly illustrates an increase in wear depth and the different wear mechanisms from the enamel to the dentin since wear behavior is closely associated with mechanical properties such as the H and E of materials tested.
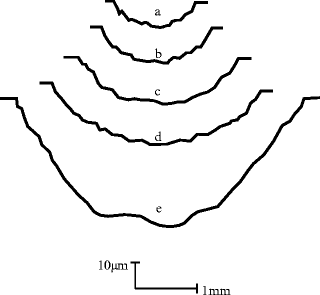
Fig. 3.4
Profile measurement on wear scars parallel to the occlusal section from the enamel to the dentin zone [19]
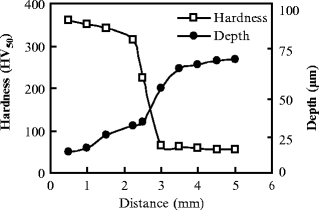
Fig. 3.5
Variations of wear depth and hardness as a function of the distance from the enamel to the dentin zone in the occlusal section [19]
In addition to the wear resistance being different between different occlusal layers of enamel for a tooth, the friction coefficient also was observed to be different between the outer and interior enamel in the occlusal section for a tooth, as shown in Fig. 3.2. Hence, it can be deduced that the friction and wear behavior of enamel change from location to location for the same tooth.
In order to explore the effect of the microstructural orientation on the tribological behavior of a human tooth, wear tests were also conducted with the same experimental parameters in the axial section of a human tooth (Fig. 3.6a). Three contact zones, located at the enamel, DEJ, and dentin, respectively, were chosen. The variations in the friction coefficient in the three contact zones are shown in (Fig. 3.6b). For the enamel zone, the friction coefficient was about 0.26 before 10 cycles, increased rapidly to 0.77 between 10 and 50 cycles, and then increased slowly to a saturation value of 0.87 after 1,200 cycles (Fig. 3.6b). In the DEJ zone, the coefficient increased rapidly at the early stage and then increased gradually to 0.97 from 10 cycles to 5,000 cycles (Fig. 3.6b). However, for the dentin zone, the variation in the coefficient fluctuated between 0.3 and 0.5 before 500 cycles, and between 0.5 and 0.85 after 500 cycles (Fig. 3.6b). The fluctuations may be attributed to the special dentin structure with a lacuna. There were more fluctuations in the axial section than in the occlusal section.
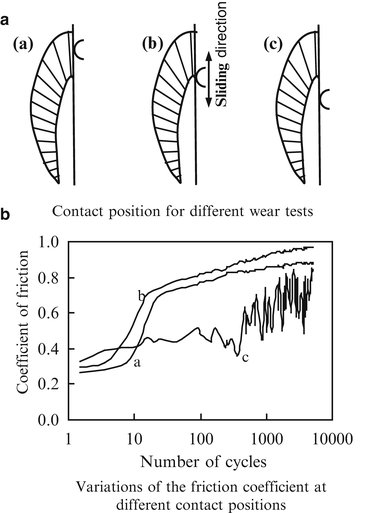
Fig. 3.6
Friction behavior of the natural tooth in the axial section [19]
In the axial section, three different types of wear scars were also obtained from the enamel to the dentin, as shown in Fig. 3.7. It can be seen that the wear morphologies are similar to those of the scars observed in the occlusal section (Fig. 3.3). The main difference is that the wear depth of the scars in the axial section is bigger than that of the scars in the occlusal section (Fig. 3.8.).
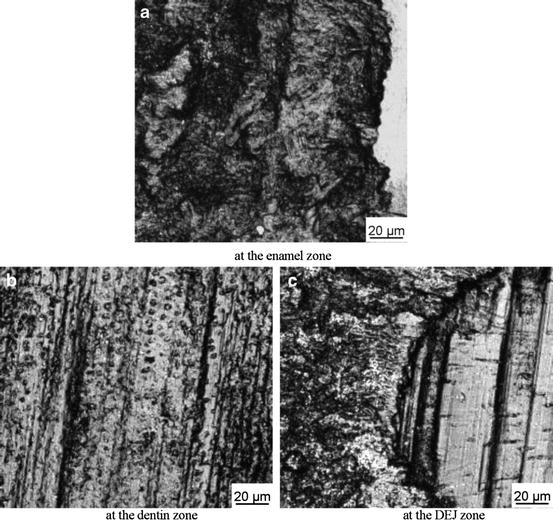
Fig. 3.7
Three types of wear scars observed in the axial section [19]
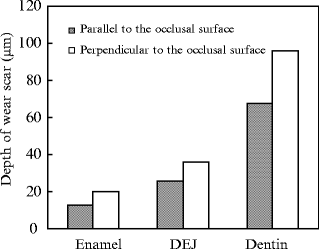
Fig. 3.8
A comparison of the wear depth between different contact zones for two different orientations [19]
Xu et al. reported a difference in the values of H and E of the enamel between the occlusal and axial sections [14]. The graphs in Figs. 3.2 and 3.6 and the bars in Fig. 3.8 demonstrate a significant difference in the friction and wear behaviors between the two orientations. First, the friction coefficient of the enamel generally appears to remain at a low value for a longer time before increasing in the occlusal section than in the axial section, as shown in Figs. 3.2 and 3.6. Second, both the depth and area of the wear scars are bigger in the axial section than in the occlusal section, as shown in Fig. 3.8. The observations imply that it is more difficult for brittle failure of enamel to occur in the occlusal section than in the axial section, which is consistent with the research conducted by Xu et al. [14]. According to Xu et al., for the occlusal section, most of the enamel rods appeared nearly perpendicular to the surface, while in the axial section, about half of the enamel rods appeared nearly parallel to the surface, and the other half appeared angled to the surface. Therefore, the anisotropic friction and wear behavior of enamel could be attributed to the enamel rods’ orientation. A similar phenomenon is observed at the dentin zone due to the effect of the dentinal tubule orientation.
In addition, some researchers have pointed out [30, 31] that due to different microstructures and mechanical properties, wear rates of enamel and dentin show a different increase tendency as the load increases. A high mineral content and corresponding hardness result in relatively low wear rates of enamel at lower loads; however, the brittle nature of enamel contributes to a high wear rate at higher loads. By contrast, dentin has a higher organic content and relative softness, which makes it less prone to fracture under oral conditions; as a result, it shows a high wear rate at lower loads but a low wear rate at higher loads. The differential wear rate between dentin and enamel occurring in areas of exposed dentin have been hypothesized to be a cofactor in the formation of some Class VI lesions [32]. Chapter 4 gives a more detailed discussion of the effect of occlusal load on the friction and wear behavior of enamel.
3.3 Process and Mechanism of Human Tooth Enamel Wear
Tooth enamel is one of those unique natural substances that still cannot be substituted for effectively by artificial restorative materials. The most important feature of enamel is its excellent wear resistance. Reportedly, sound enamel under friction from mastication and biting lost only a 10–40-μm-thick layer per year [33]. Mass [34] pointed out that the variation in crystallite orientation of prismatic enamels may contribute to optimal dental function through the property of differential wear in functionally distinct regions of teeth. In this section, we report on our detailed study on the wear behavior of human tooth enamel [35]. Tests lasting up to 10, 100, 1,000, 2,000, and 5,000 cycles were conducted, respectively. The main objective was to understand the process and mechanism of enamel wear.
Flat enamel specimens were prepared using the method mentioned in Sect. 3.2. Only 0.2–0.3 mm of each specimen was ground and polished off to obtain a surface similar to the original occlusal surface of enamel in the mouth. Due to its excellent biocompatibility, pure titanium (TA2) and titanium alloy (TC4) have often been used as dental materials in recent clinical practice. Compared with TA2 (240 HV50g), the Vickers hardness of TC4 (350 HV50g) was closer to that of human tooth enamel (360 HV50g). In terms of the evolution and stable value of the friction coefficient and the worn surface morphology and wear scar depth, the enamel–titanium alloy pair was similar to the enamel–enamel pair [36]. Thus, a TC4 ball with a 40-mm diameter was chosen as a counterpart in this study. The composition of TC4 is C 0.043 %, Al 6.020 %, H 0.011 %, O 0.160 %, V 4.100 %, Fe 0.168 %, and Ti the balance. The mechanical properties and surface roughness of specimens are shown in Table 3.2.
Table 3.2
Mechanical parameters and surface roughness of enamel and TC4
Specimen
|
Tensile strength (GPa)
|
Elastic modulus (GPa)
|
Poisson ratio
|
Hardness (HV50)
|
Roughness (μm)
|
---|---|---|---|---|---|
Enamel
|
0.06
|
94
|
0.28
|
360
|
0.20
|
TC4
|
0. 62
|
113
|
0.30
|
350
|
0.40
|
A normal force of 20 N, a reciprocating amplitude of 500 μm, and a frequency of 2 Hz were used for all the tests. The composition of artificial saliva is listed in Table 3.1.
For the wear tests lasting up to 5,000 cycles, typical variations in the friction coefficient, as a function of the number of cycles, are shown in Fig. 3.9. A lower friction coefficient lasted for about 20 cycles, increased to 0.8 after 100 cycles, and then increased slowly to a saturation value of 0.91 after 2,000 cycles.
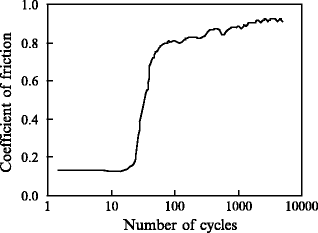
Fig. 3.9
Variation in the friction coefficient with cycles subjected to 5,000 cycles [35]
Wear scars on the enamel surface for different cycles were first examined by laser confocal scanning microscope (LCSM), and the typical micrographs are shown in Fig. 3.10. Figure 3.11 gives the typical scanning electronic microscopy (SEM) micrographs of the central area of wear scars. Energy dispersive X-ray (EDX) spectra of wear particles on enamel surfaces subjected to different cycles are shown in Fig. 3.12.
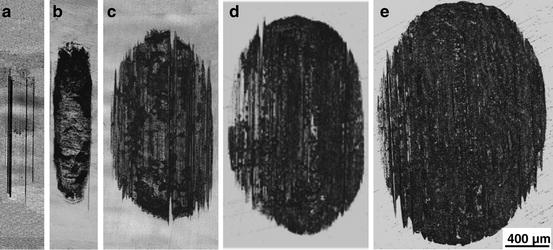
Fig. 3.10
LCSM micrographs of enamel wear scars subjected to different cycles [35]: (a) 10 cycles; (b) 100 cycles; (c) 1,000 cycles; (d) 2,000 cycles; (e) 5,000 cycles
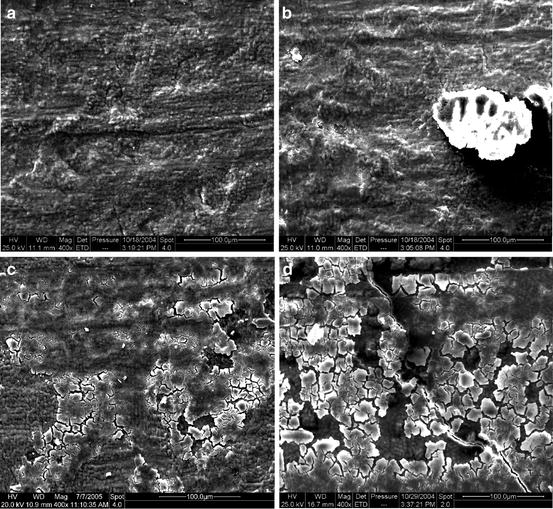
Fig. 3.11
SEM micrographs of wear scars on the surfaces of enamel subjected to different cycles [35]: (a) 100 cycles; (b) 1,000 cycles; (c) 2,000 cycles; (d) 5,000 cycles
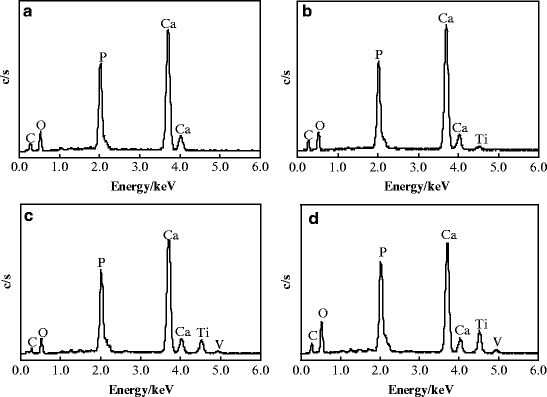
Fig. 3.12
EDX spectra of wear particles on enamel surfaces subjected to different cycles [35]: (a) original surface; (b) 1,000 cycles; (c) 2,000 cycles; (d) 5,000 cycles
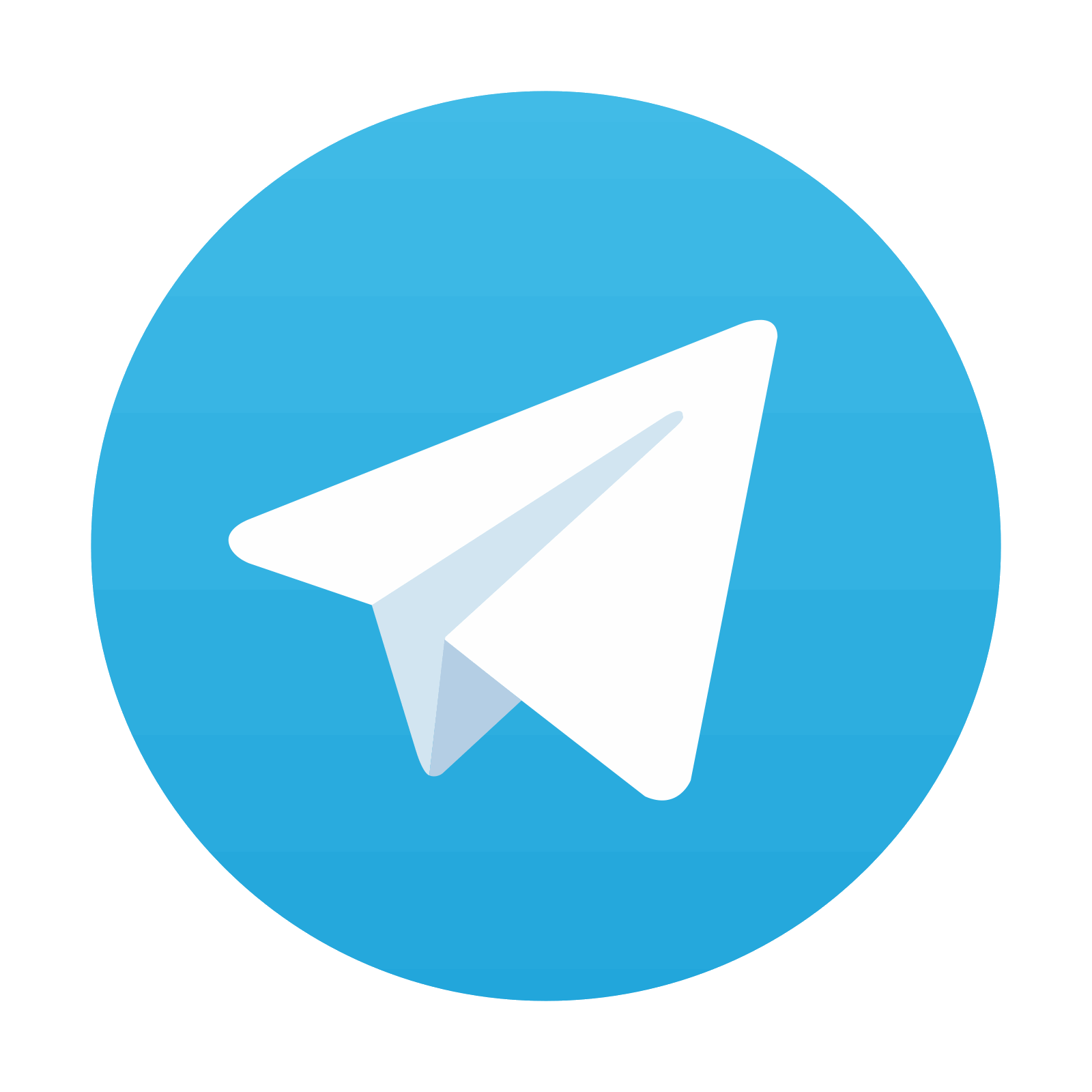
Stay updated, free dental videos. Join our Telegram channel

VIDEdental - Online dental courses
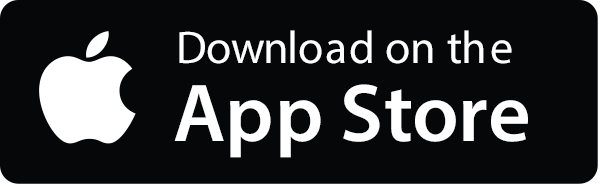
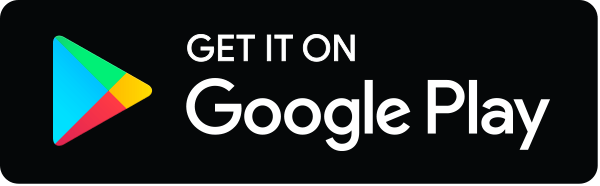