Abstract
Objectives
Light-cured composites undergo shrinkage during polymerization. The aim of this study was to evaluate regional shrinkage within a light-cured composite during polymerization by microcomputed tomography and mechanical properties by nanoindentation in bonded or non-bonded class-I cavity.
Methods
Zirconium oxide spherical fillers (30 μm diameter) were added as markers to a composite resin, filled into a box-shaped class I cavity with or without a bonding agent. The marker fillers were traced in 3D scans obtained by micro-CT before and after polymerization using a software (TRI/3D-BON). The average hardness of the resin composites determined by nanoindentation at each 250 μm depth was plotted against depth.
Results
In the bonded cavity, the filler particles at the top region moved toward the bottom of cavity, but at deeper depths, the direction of vertical movement changed toward the top of cavity (irradiated surface). A significant linear regression was found between filler displacement and composite depth ( R 2 = 0.9761). In the unbounded cavity, all the fillers moved toward the light curing source, and a significant power-law regression was found between filler displacement and composite depth ( R 2 = 0.849). In both groups, the data scattering increased at regions deeper than 3.5 mm, where the hardness, representing degree of conversion of composite, significantly decreased compared to the surface region.
Significance
The magnitude and direction of regional polymerization shrinkage depends on boundary conditions, depth and conversion degree. Polymerization shrinkage effect is most significant at the deepest part of the cavity. The application of micro-CT combined with sophisticated image analysis is a novel approach to investigate shrinkage mechanisms of dental composites.
1
Introduction
Current dental composites consisting of inorganic fillers and a polydimethacrylate matrix are polymerized by rapid free-radical reactions activated by visible light in situ. These biomaterials are becoming increasingly popular, and preferred over metals for direct restorations . However, currently available dental restorative composites undergo dimensional shrinkage when they are polymerized . The volume loss during the rapid polymerization generates stresses that have been recognized as important factors affecting the marginal integrity of the composite restorations, leading to debonding from the surrounding tooth structure, and formation of gaps .
Interfacial gap and residual stresses greatly affect the longevity and success of a restoration. Therefore, it is necessary to understand the shrinkage mechanism and strategies to overcome or prevent the detrimental effects .
The polymerization reaction within a light-cured bulk composite paste varies with depth. The variation is a result of gradual loss of power density deeper inside resin, leading to a slower polymerization reaction for the deeper parts . It was broadly agreed that light-curing resin composites shrink toward the direction of the light-source . In this regard, it was suggested that the polymerization of a composite filled into a boxed-shaped (class I) cavity and light-cured from above, would start from superficial area of the composite, and the direction of shrinkage propagation was oriented toward the light source, resulting in shrinkage-generated gaps along the cavity floor . However, hypotheses about contraction patterns of light-curing dental composites are still somewhat controversial.
Shrinkage vectors represent amount and direction of the composite movement during polymerization. The effects of shrinkage have been widely investigated by techniques that require specimen cutting, such as evaluating the gap formation at the cavity floor, composite mechanical properties or bond strength; meanwhile, direct measurement of shrinkage attributes of composite in a cavity has proved to be technically difficult .
Finite element methods have been utilized as numerical techniques to investigate theories suggested about shrinkage kinetics, effect of light direction and stress development in modeled cavities . In addition, high-resolution topographic techniques have enabled nondestructive assessment of the internal structures of biomaterials. Using these techniques, monitoring polymerization shrinkage is possible if the structural features at each spatial location are precisely traceable. Microfocus X-ray computed tomography (micro-CT), is a high-resolution three-dimensional (3D) imaging technique that has been broadly accepted in research on biomedical structures, including dental composites .
Inai et al. first proposed the idea of tracing fillers in a composite material using micro-CT imaging . More recently, Chiang et al. used more sophisticated analyses of micro-CT images for tracing marker fillers before and after polymerization in a composite, and elaborated on shrinkage vectors in a flowable composite .
Development of such computer-aided quantitative techniques is essential to gain a deeper understanding of polymerization shrinkage and minute details of polymerization mechanism for various dental composites. Recent advances in computer software have enabled achieving new insights into polymerization of composites using 3D micro CT imaging.
It has been reported that mechanical properties, curing rate and the boundary conditions significantly affect the polymerization shrinkage, but to date, few studies have attempted to experimentally evaluate all factors in a simulated cavity setup.
Therefore, our aim in the current study was to assess local axial polymerization shrinkage of a universal light-cured resin composite filled into a class-I cavity with or without bonding to cavity walls, in relation to nanoindentation hardness. For this purpose we developed a filler movement tracing method for 3D analysis of the micro-CT image. The null hypothesis was that boundary conditions did not affect local axial polymerization shrinkage of a light-cured composite in a class-I cavity.
2
Materials and methods
2.1
Materials
2.1.1
Experimental resin composite
An experimental universal composite was developed for this study (Tokuyama Dental, Tokyo, Japan). Radio-opaque spherical zirconia fillers 30 μm in diameter were added to the composite as marker fillers to be traced by micro-CT imaging. Composition of the experimental material is detailed in Table 1 . Fig. 1 schematically illustrated the experimental procedure.
Composition | Weight % |
---|---|
Bis-GMA | 10.2 |
TEGDMA | 6.8 |
Silica–zirconia filler (0.4 μm diameter) | 56.3 |
Silica–titania filler (0.1 μm diameter) | 24.2 |
Zirconia marker fillers (30 μm diameter) | 2.5 |
Others (initiator, stabilizer, pigment, etc.) | <1 |
2.1.2
Cavity preparation
Two composite blocks each with a class I cavity were prepared from a hybrid resin composite (Solare, GC Corp., Tokyo, Japan). For this purpose, a box-shaped cavity (5 mm × 5 mm × 5 mm) was made in an acrylonitrile butadiene styrene (ABS) plastic resin as the model block. Then, an impression of the model block was taken using putty and regular type hydrophilic vinyl polysiloxane impression material (Exahiflex, GC Corp.). Two replicas of the model block were fabricated by incremental filling of the impression mold with Solare composite. Each layer was light-cured for 40 s, and the whole block was finally cured with a laboratory light-curing unit (α-light II, J.MORITA, Tokyo, Japan) for 5 min to ensure complete polymerization of the resin composite.
The cavities were ultrasonically cleansed in acetone for 5 min, and the composite blocks were stored for a week in water.
2.1.3
Filling procedures
In the bonded group, a primer mixed with a silane coupling agent (Clearfil Porcelain Bond Activator and Clearfil SE Bond primer, Kuraray Medical, Tokyo, Japan) was applied to the cavity walls and air-dried for 5 s. A bonding agent was then placed on the primed surface and irradiated for 10 s.
In the unbonded group, the cavity walls were treated with white petrolatum (White Vaseline, Kenei Pharmaceutical Co., Osaka, Japan) as a separating agent.
In both groups, the cavity was filled in bulk using the experimental composite material. Each cavity was covered with a transparent cellulose strip and a microscopic cover slip to level off the surface and remove the residual material. The block was then immediately placed in micro-CT imaging chamber and scanned before light curing, as explained later in this section. Then composite was photo-polymerized under 60 s continuous exposure to an LED light-curing unit (Dentaport, Morita Mfg. Corp., Kyoto, Japan) at 600 mw/cm 2 light intensity. The experimental resin composite was cured while the sample was fixed to the stage in the chamber. All samples were rescanned after photo-polymerization using the micro-CT scanner.
2.2
Microfocus X-ray computed tomography
The operating conditions for the microfocus X-ray computed tomography (micro-CT) device (TDM-1000, Yamato, Tokyo, Japan) were100 kV voltage and 50 μA current, and 14.2 μm voxel dimensions. The composite pastes were packed into the cavities as explained earlier, and fixed upright with double-faced tape at marked positions on the micro-CT sample holder. The data before and after polymerization were subjected to image segmentation and registration to trace the movement of the marker fillers during polymerization.
2.3
Filler movement tracing software
Acquired scan data of composite before and after polymerization were imported into 3D-BON software (Ratoc Systems Engineering, Tokyo, Japan). The scales of the images were set according to the voxel size of the micro-CT data. From each cavity, serial 2D images were stacked to form 3D images. The 3D images before and after polymerization were obtained under the same conditions, and were therefore already aligned. The composite volume was then digitally cropped from each 3D image. After deciding the origin of coordinates of the 3D image, marker fillers were abstracted throughout the volume by a segmentation procedure as summarized here;
A noise reducing filter was applied to the data, to remove bright pixels that were too small to be marker filler. Following removing the noise, a threshold value was determined locally on (4 × 4 × 4) matrices within the 3D images, on which basis each image was converted from the gray scale into binary images. The result included two elements (two voxel types); marker fillers and matrix background.
In order to trace the filler movement, it was necessary to recognize each filler after polymerization and pair it with the same particle in the image before polymerization.
A cluster-labeling algorithm was used to extract the fillers and perform this pairing procedure; it was considered that the filler movement distance would be within 140 μm (around 10 voxels) and the volume error of each particle would be less than 100%, equivalent to approximately 25% error in radius.
Movement distance of each filler after polymerization would be then obtained in each direction ( x , y , z ).
2.4
Nanoindentation analysis
In order to evaluate the hardness of the composites at each depth, the samples were subjected to nanoindentation. A 2 mm-thick slice was obtained from the polymerized composite after micro-CT, by cross-sectioning the block along the central axis using a low-speed diamond saw under water cooling (Isomet, Buehler, Lake Bluff, IL, USA).
The cross-sectional surface was then fine polished by SiC papers and diamond pastes down to a particle size of 0.25 μm to produce a smooth surface suitable for nanoindentation. Nanoindentation hardness was then measured at a maximum force of 50 mN reached at 1 mN/s loading rate at 20 μN increments by a Berkovich indenter attached to a nanoindentation device (ENT-1100a, Elionix, Tokyo, Japan). Hardness was calculated by dividing the maximum force by the projected area as previously described . The indentations were made from the top toward the bottom of the cavity in a 10 × 20 matrix, with 10 indentations at every 0.25 mm depth. At each depth, the indentations were 0.4 mm apart from each other. To avoid data from indentations performed on the zirconia marker fillers rather than the resin matrix, the shape of indentation, the load–displacement curve and range of hardness data were closely inspected. Occasionally hardness values that were out of range (too high) were excluded from the analysis.
2.5
Statistical analysis
In order to present average filler movement distance against depth, filler movement data from each cavity after polymerization was divided to approximately 165 regions in the top-to-bottom direction. The movement distance of fillers along z -axis at each region was averaged, and the results were plotted against cavity depth, and subjected to regression analysis ( Fig. 2 ).
Likewise, the hardness was plotted against cavity depth. Two-way ANOVA with depth and boundary condition as two factors was used to analyze the factors. Within each material, one-way ANOVA was used to detect the depth at which the hardness showed significant decrease compared to the hardness at the top 2 mm of the cavity by Dunnett test. Statistical significance was defined as p < 0.05.
2
Materials and methods
2.1
Materials
2.1.1
Experimental resin composite
An experimental universal composite was developed for this study (Tokuyama Dental, Tokyo, Japan). Radio-opaque spherical zirconia fillers 30 μm in diameter were added to the composite as marker fillers to be traced by micro-CT imaging. Composition of the experimental material is detailed in Table 1 . Fig. 1 schematically illustrated the experimental procedure.
Composition | Weight % |
---|---|
Bis-GMA | 10.2 |
TEGDMA | 6.8 |
Silica–zirconia filler (0.4 μm diameter) | 56.3 |
Silica–titania filler (0.1 μm diameter) | 24.2 |
Zirconia marker fillers (30 μm diameter) | 2.5 |
Others (initiator, stabilizer, pigment, etc.) | <1 |
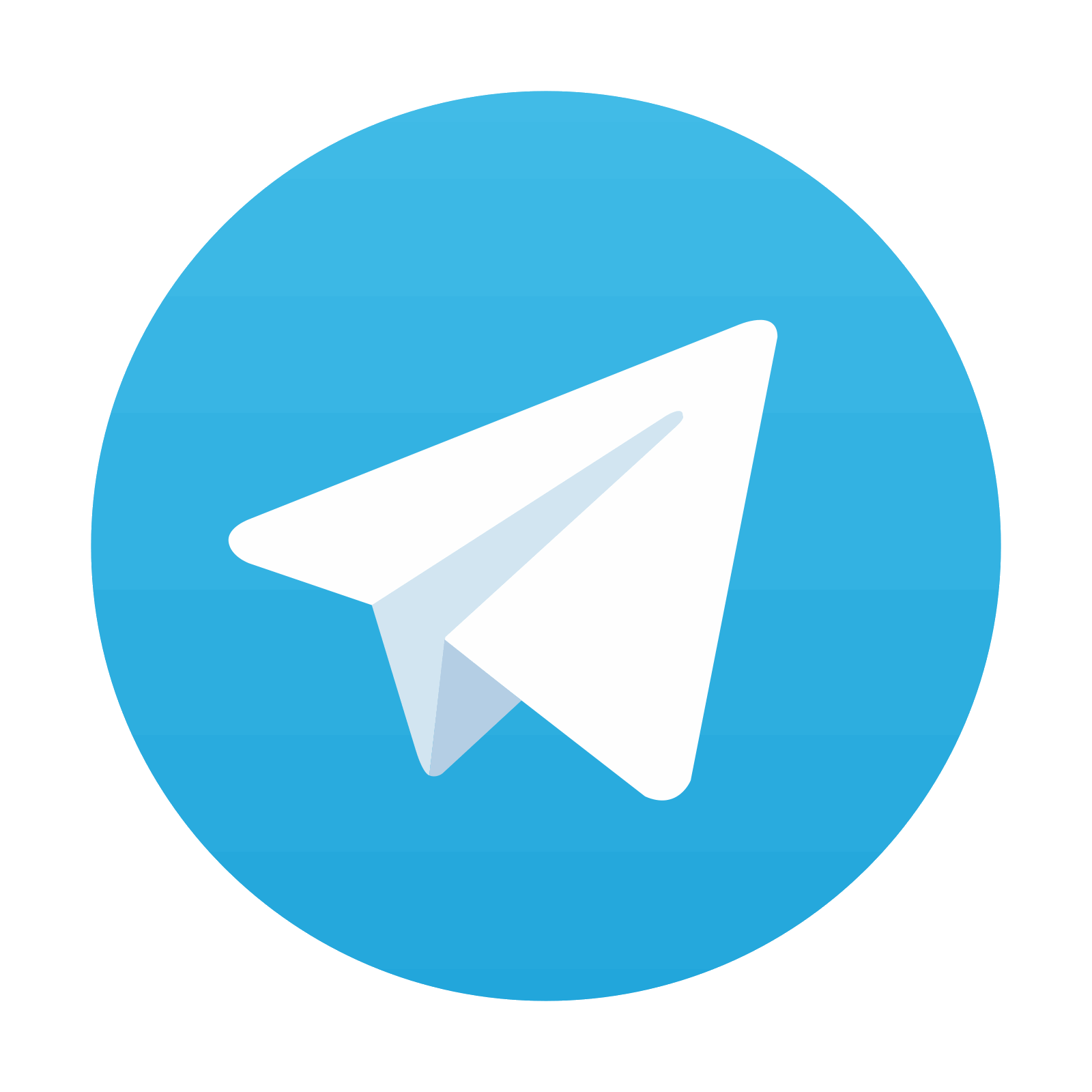
Stay updated, free dental videos. Join our Telegram channel

VIDEdental - Online dental courses
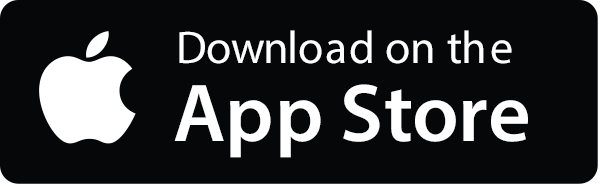
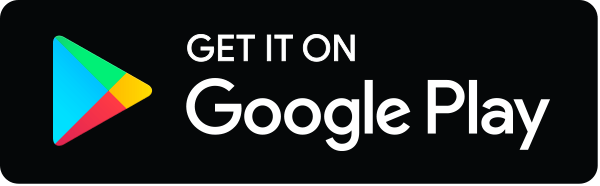
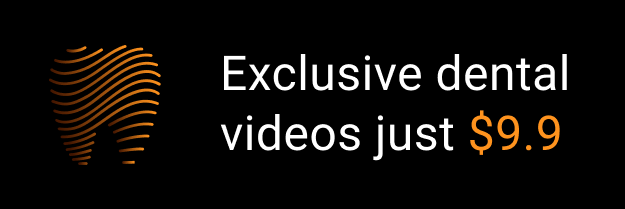