Abstract
Objective
Adding antimicrobial/anti-MMP quaternary ammonium methacrylates (QAMs) to comonomer blends should not weaken the mechanical properties of dental resins. This work evaluated the degree conversion and mechanical properties of BisGMA/TEGDMA/HEMA (60:30:10) containing 0–15 mass% QAMs A–E (A: 2-acryloxyethyltrimethyl ammonium chloride; B: [3-(methacryloylamino)propyl]trimethylammonium chloride; C: [2-(methacryloxy)ethyl] trimethyl ammonium chloride; D: diallyldimethyl ammonium chloride; E: 2-(methacryloyloxy) ethyltrimethyl ammonium methyl sulfate.
Methods
Unfilled resins with and without QAM were placed on ATR-FTIR and light-polymerized for 20 s in a thin film at 30 °C. Unfilled resin beams were casted from square hollow glass tubings. Half of the beams were tested after 3 days of drying (control); the other half were tested wet after 3 days of water storage.
Results
Addition of QAMs in control resins significantly increased conversion 600 s after light termination, with the exception of 5% MAPTAC ( p < 0.05). Increase of QAM content within a formulation significantly increased conversion. Control beams gave dry Young’s moduli of ∼700 MPa. Addition of 5, 10 or 15 mass% QAMs produced significant reductions in dry Young’s moduli except for 5% B or C. 15 mass% A, B and C lowered the wet Young’s moduli of the resin beams by more than 30%. The ultimate tensile stress (UTS) of control dry resin was 89 ± 11 MPa. Addition of 5–10 mass% QAMs had no adverse effect on the dry UTS. After water storage, the UTS of all resin blends fell significantly ( p < 0.05), especially when 15 wt% QAMs was added. Control dry beams gave fracture toughness ( K IC ) values of 0.88 ± 0.1 MPa m 1/2 . Wet values were significantly higher at 1.02 ± 0.06 ( p < 0.05). K IC of dry beams varied from 0.85 ± 0.08 at 5% QAMs to 0.49 ± 0.05 at 15% QAMs. Wet beams gave K IC values of 1.02 ± 0.06 MPa m 1/2 that fell to 0.23 ± 0.01 at 15% QAMs.
Significance
Addition of 10% QAMs increased the degree of conversion of unfilled resins, but lowered wet toughness and UTS; addition of 15% QAMs lowered the mechanical properties of wet resins below acceptable levels.
1
Introduction
Quaternary ammonium methacrylates (QAMs) have been added to dental comonomer blends for their antibacterial and anti-MMP activity . Because most QAMs are monomethacrylates, it is important to determine if addition of QAMs to comonomer blends cause any weakening of their mechanical properties, and if so, at what concentration. A good example of a QAM used in a dental product is 12-methacryloyloxydodecylpyridinium bromide (MDPB). It is used in Clearfil SE Protect as an antibacterial primer .
It is well-known that water sorption into hydrophilic polymers plasticizes them and lowers their mechanical properties . Unfilled resins tend to have low mechanical properties values because they have no reinforcing fillers. The lower flexural strength and flexural modulus of unfilled resins results in a lower fracture toughness , giving them values similar to compomers .
As one incorporates any monomethacrylate into comonomer blends at the expense of dimethacrylates, the degree of cross-linking of the polymer decreases. This results in lower flexural strength and flexural modulus. Presumably, this should also lower other mechanical properties of resins.
The purpose of this study was to determine if addition of 5, 10 or 15 wt% QAMs to a BisGMA/TEGDMA/HEMA blend would lower the degree of conversion or mechanical properties of beam specimens of unfilled resins. The first null test hypotheses was that (1) addition of 5, 10 or 15 wt% QAMs has no effect on the degree of conversion or dimethacrylate blends, (2) addition of 5, 10 or 15 wt% QAMs has no effect on the mechanical properties of dimethacrylate blends, (3) that addition of 5, 10 or 15% QAMs to dimethacrylate blends immersed in water does not produce water-sorption induced changes in the mechanical properties of QAM-containing dimethacrylates.
2
Materials and methods
2.1
Degree of conversion
Before testing the effect of QAM-containing adhesives on the mechanical properties of unfilled resins, a careful investigation of the effect of incorporation of QAMs into dental adhesives is necessary to verify their role in polymerization. Indeed, it was clearly shown that the degree of conversion (DC) of dental adhesives is an important parameter since low mechanical properties associated with monomer elution are related with low percentage of monomer to polymer conversion within resin-based materials .
Previous investigations showed that DC depends on resin type, hydrophilicity, solvent content and photo-initiator blended within the monomers. It has been shown that even though camphoroquinone (CQ) is the most common photo-initiating system in dental resin restorative materials , it may impair the polymerization of hydrophilic monomers due to CQ’s intrinsic hydrophobic nature. On the other hand, the use of CQ-alternative photoinitiators, such as diphenyl(2,4,6-trimethylbenzoyl)-phosphine oxide), TPO, may improve the extent of polymerization (Ep) of hydrophilic adhesive formulations .
One drop of each resin mixture was placed between two, 0.15-mm thick, laterally placed microscope cover glass plates acting as shims, directly on the surface of a diamond crystal in a horizontal attenuated total reflectance heated unit (25 °C) stage (Golden Gate Mk II, SPECAC Inc., Cranston, RI) using a 1 mL disposable syringe (Norm-Ject, Tuttlingen, Germany). The attachment was positioned in the optical compartment of a Fourier transform infrared spectrophotometer (FTS-40, Digilab/BioRad, Cambridge, MA). A 1.5 cm × 1.5 cm × 76 μm piece of polyester film (Mylar, Type D, Polymer Plastics Corporation, Reno, NY, USA) was immediately placed over the top of the deposited resin (covering the two glass shims laterally) to exclude oxygen ( Fig. 1 ), and a 3-mm thick, clear glass plate was positioned over the top of the assembly and pressed down, forcing the fluid to spread and attain the final thickness of the two lateral glass shims. The flat end of the light guide of a quartz-tungsten-halogen light-curing unit (Optilux 401, Demetron/Kerr, Danbury, CT) was placed coincident with the underlying diamond element, and directly against the upper glass surface. The irradiance of the curing light was measuring through the same 3-mm thick glass plate, using a laboratory grade spectroradiometric system, consisting of a 6″ NIST-traceable integrating sphere (Labsphere, North Sutton, NH) and a spectrometer (USA2000, Ocean Optics, Dunedin, FL) to be 525 mW/cm 2 . The resins were exposed to blue light for 20 s and spectra were obtained continuously until 605 s from droplet application. The experimental setup simulated dispensing of an adhesive resin in a thin layer on a tooth prepared surface clinically, but without air-drying ( Fig. 1 ).
In addition, this experimental set-up is similar to that used to measure polymerization shrinkage with the Watts Bonded Disc apparatus, allowing conversion data to ultimately by correlated with shrinkage values (not presented in the current work). Kinetic infrared (IR) spectra were obtained between 4000 and 700 cm −1 at 2 cm −1 resolution at one scan per second, with no averaging. Spectral acquisition was initiated immediately upon resin droplet deposition to obtain the IR spectra of each solution group in the uncured state and the curing light was activated 5 s after droplet deposition.
Preliminary FTIR spectra of one QAM monomer (MAPTAC) revealed an absorption band at 1653 cm (circled in Fig. 2 B ) that interfered with the use of the conventional method of determining monomer conversion of methacrylate-based dental resins. That method uses the ratio of aliphatic C C absorption (1636 cm −1 ) to that of the internal standard aromatic C C band (1608 cm −1 ) in the cured and uncured state and relies on the absence of any other peak within the range of analysis ( Fig. 1 ) . MAPTAC’s spectrum, not allowing the conventional spectral analysis to be performed to determine monomer conversion. Comparison of spectral absorbance of representative resins in the unhydrogenated and hydrogenated states confirms the presence of an interfering peak at 1653 cm −1 in MAPTAC, even after total removal of the aliphatic C C groups ( Fig. 3 ). In Fig. 3 , the left panel shows the absence of the C C group at 1636 cm −1 in the MCMS resin following hydrogenation. The right panel shows the total loss of the 1636 cm −1 C C group in MAPTAC following hydrogenation but the residual presence of an interfering absorption band at 1635 cm −1 that prevents conventional method of spectral analysis to be used to determine monomer conversion in this frequency range.
Thus, the extent of polymerization (Ep) was determined by comparing changes in the loss of absorbance of a relatively isolated peak at 1318 cm −1 , that represents the trans p (=CH)s in-plane CH deformations . The method used to determine conversion using 1318 cm −1 absorption peak was that of determining the absorption of the peak height with respect to a baseline drawn between the troughs in either side of the peak ( Fig. 4 ).
IR spectra showing the method for determining the peak absorbance height at 1318 cm −1 in the uncured (upper) and cured (lower) states are shown in Fig. 5 .
Verification that the 1318 cm −1 peak was indeed measuring C C, absorbance was made by comparing the IR spectra of unhydrogenated and hydrogenated Scotchbond Multi-Purpose resin. Total absence of the 1318 cm −1 peak confirmed identity of the functional group associated with this peak ( Fig. 5 ).
Comparison of unhydrogenated Bis-GMA/TEGDMA-based adhesive (SBMP) upper scan, showing the presence of a high absorption located in 1318 cm −1 and the total absence of this peak at that wavenumber, following hydrogenation of the C C (lower line).
Prior to utilization of the 1318 cm −1 peak, conversion of a Bis-GMA/TEGDMA-based dental resin (SBMP) was compared using the conventional method, and that when using the 1318 cm −1 peak, to show that no significant differences were found ( Fig. 6 ).
Monomer conversion values were determined for each second following light initiation, and conversion at specific times following light initiation were grouped: 20, 40, 60 and 600 s. Five repetitions for each test condition were made ( Fig. 6 ).
Comparison of real-time monomer conversion profiles for SBMP using the conventional 1636 cm −1 /1608 cm −1 ratio technique (lower line) and that using change in the absorption peak located at 1318 cm −1 (upper line, Fig. 6 ).
The rate of cure was obtained by calculating the first derivative of the smoothed conversion curve with respect to time, using data-analysis software (Logger Pro 3.5, Vernier Software & Technology, Beaverton, OR). The maximum polymerization rate (expressed as DC%/s) was obtained during the first 20 s of exposure.
2.2
Statistical analysis
One-way ANOVAs were used to evaluate how resin formulation affected the maximum rate of conversion and extent of the DC 600 s following light initiation, as measured by FTIR. Differences between groups were calculated using Tukey’s post hoc test. Statistical significance was preset at α = 0.05.
2.3
Mechanical properties of resins
The control resin blend was 60 wt% BisGMA, 30% TEGDMA, 9% HEMA 1.0% [diphenyl](2,4,6-trimethylbenzoyl)-phosphine oxide (TPO). It was used without solvent. The QAMs were: ATA = 2-acryloxyethyltrimethylammonium chloride; METMAC = [3-(methacryloylamino)propyl]trimethylammonium chloride; DDAC = diallyldimethylammonium chloride; MCMS = methacryloylcholine methyl sulfate; MAPTAC = [2-(methyloyloxy)ethyl]trimethylammonium chloride. When 5, 10 or 15 wt% QAMs were added to the control blend, the BisGMA was reduced an equal amount.
The beams used for fracture toughness were made in a stainless steel mold with internal dimensions of 24 mm in length, 5 mm in height, 2.5 mm width, with a razor blade incorporated into the mold 12 mm from either end, projecting 2.5 mm into the empty mold space. The mold was placed on a glass plate covered with Mylar film. After the mold was filled with neat comonomers, the filled mold was covered by another Mylar film and then a glass slide. The mixture was exposed to a Demitron dental light (600 mW/cm 3 ) for 40 s, overlapping the diameter of the light-guide down the length of the beam for 4 exposures. Then the glass plates were removed and the light-curing repeated on the other side. After disassembling the molds, the polymerized beams were carefully removed laterally to avoid damage to the preformed notch. Twenty beams were made of each resin ( Table 1 ). Each group was randomly divided into “dry” and “wet” groups. Dry beams were stored dry for 3 days at 37 °C prior to testing. All beams were sanded with dry 2000 grit SiC abrasive paper to remove any irregularities and to make them perfectly flat.
QAM | Composition of comonomer blends | Max cure rate (%/s) | DC 20 s | DC 40 s | DC 60 s | DC 600 s |
---|---|---|---|---|---|---|
5% ATA | 5% ATA/52.75% BisGMA/30% TEGDMA/10% HEMA/1.0% TPO/1.25% H 2 O | 18.2 ± 0.7 B,C,D | 72.3 ± 0.4 | 76.3 ± 1.1 | 79.5 ± 0.0 | 84.4 ± 0.2 E,F |
10% ATA | 10% ATA/46.5% BisGMA/30% TEGDMA/10% HEMA/0.0% TPO/2.5% H 2 O | 18.8 ± 0.8 A,B,C | 75.4 ± 2.3 | 81.1 ± 0.9 | 82.4 ± 1.0 | 89.1 ± 0.1 B,C,D |
15% ATA | 15% ATA/40.25% BisGMA/30% TEGDMA/10% HEMA/1.0% TPO/3.75% H 2 O | 19.9 ± 0.7 A | 79.2 ± 1.4 | 82.4 ± 0.6 | 83.7 ± 0.5 | 90.4 ± 0.3 A,B |
5% MAPTAC | 5% MAPTAC/49% BisGMA/30% TEGDMA/10% HEMA/10% TPO/5.0% H 2 O | 15.1 ± 1.0 F | 65.9 ± 3.7 | 69.7 ± 5.1 | 71.9 ± 4.6 | 78.5 ± 3.9 G,H |
5% DDAC | 5% DDAC/51.3% BisGMA/30% TEGDMA/10% HEMA/10% TPO/2.7% H 2 O | 18.2 ± 1.2 B,C,D | 76.8 ± 1.9 | 81.4 ± 1.5 | 82.1 ± 2.0 | 90.2 ± 0.5 A,B,C |
5% MCMS | 5% MCMS/52.75% BisGMA/30% TEGDMA/10% HEMA/10% TPO/1.25% H 2 O | 17.4 ± 0.5 C,D,E | 69.9 ± 0.6 | 73.4 ± 0.6 | 74.6 ± 0.8 | 81.5 ± 1.5 F,G,H |
10% MCMS | 10% MCMS/46.5% BisGMA/30% TEGDMA/10% HEMA/1.0% TPO/2.5% H 2 O | 17.2 ± 0.2 D,E | 72.6 ± 2.7 | 76.7 ± 2.7 | 78.0 ± 2.6 | 85.8 ± 2.2 D,E |
15% MCMS | 15% MCMS/40.25% BisGMA/30% TEGDMA/10% HEMA/1.0% TPO/3.75% H 2 O | 18.1 ± 0.7 B,C,D | 79.6 ± 1.2 | 83.9 ± 0.9 | 85.3 ± 0.9 | 91.5 ± 1.2 A |
5% METMAC | 5% METMAC/52.75% BisGMA/30% TEGDMA/10% HEMA/1.0% TPO/1.25% H 2 O | 17.0 ± 0.1 D,E | 68.1 ± 1.9 | 71.8 ± 1.8 | 73.7 ± 0.9 | 78.9 ± 0.9 G,H |
10% METMAC | 10% METMAC/46.5% BisGMA/30% TEGDMA/10% HEMA/1.0% TPO/2.5% H 2 O | 17.3 ± 0.7 D,E | 70.8 ± 1.2 | 75.0 ± 0.5 | 76.4 ± 0.6 | 81.8 ± 0.5 F,G |
15% METMAC | 15% METMAC/3 = 40.25% BisGMA/30% TEGDMA/10% HEMA/1.0% TPO/3.75% H 2 O | 18.9 ± 0.5 A,B | 77.2 ± 0.5 | 80.6 ± 0.9 | 81.8 ± 0.8 | 87.4 ± 1.0 C,D,E |
Control | 60% BisGMA/30% TEGDMA/10% HEMA/1.0% TPO | 16.4 ± 0.7 E | 65.6 ± 1.9 | 70.6 ± 0.9 | 72.1 ± 0.9 | 78.3 ± 02.2 H |
SBMP | 60% BisGMA/40% HEMA/1% DPIHP (Guo et al., 2007) | 6.5 ± 0.3 G | 51.7 ± 0.4 | 56.9 ± 1.1 | 59.4 ± 0.6 | 66.2 ± 0.6 I |
“Wet” beams were placed in distilled water for 3 days at 37 °C prior to testing. All beams were tested on the same rounded supports 22 mm apart. The dry beams were tested in air. The wet beams were tested under water at a compressive cross-head speed of 0.1 mm/min. The universal testing machine used a 22 N load cell to measure load. Displacement was measured from the cross-head movement. The exact notch depth (a) was measured with a microscope but was nominally 2.5 mm. K IC was calculated as:
K IC = 3 ( a / w ) 1 / 2 [ 1.99 − a / w ( 1 − a / w ) ( 2.15 − 3.93 a / w + 2.7 ( a / w ) 2 ] P S 2 ( 1 + 2 a / w ) ( 1 − a / w ) ( 1 − a / w ) 3 / 2 B W 3 / 2
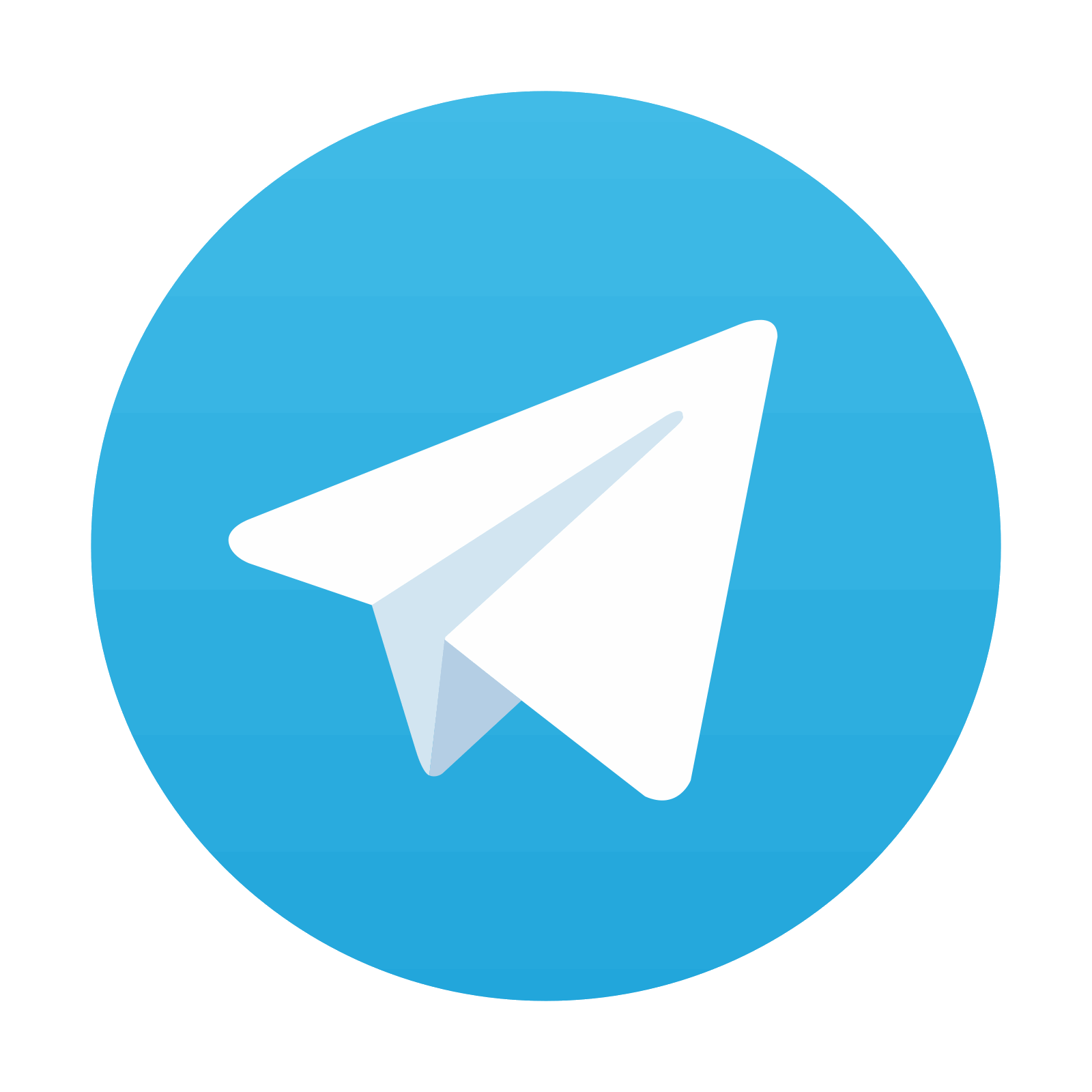
Stay updated, free dental videos. Join our Telegram channel

VIDEdental - Online dental courses
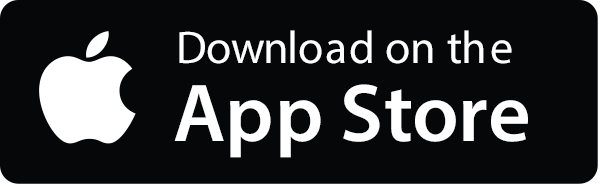
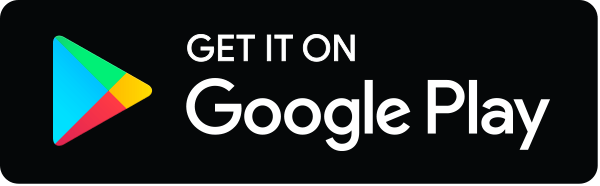