Introduction
Drugs that block the renin-angiotensin system (RAS) are widely used for treating hypertension, heart and kidney failure, and the harmful effects of diabetes. Components of the RAS have been identified in various organs, but little is known of their effects on bone remodeling. The aim of this study was to evaluate whether the blockage of the RAS influences strain-induced bone remodeling in a model of orthodontic tooth movement.
Methods
An orthodontic appliance was placed in C57BL6/J mice that were randomly divided into 2 groups: vehicle-treated mice (VH) and mice treated with losartan (an angiotensin II receptor blocker). Orthodontic tooth movement and the number of tartrate-resistant acid phosphatase-positive cells were determined by histopathologic analysis. The expression of mediators involved in bone remodeling was evaluated by quantitative real-time polymerase chain reaction. Blood pressure was measured before and during the experimental period.
Results
Orthodontic tooth movement and tartrate-resistant acid phosphatase-positive cells were significantly reduced in the losartan group compared with the VH group. mRNA levels of osteoclast markers (RANK, RANKL, cathepsin K, and metalloproteinase 13) were lower in the losartan mice than in the VH group, whereas the expressions of osteoblast markers and negative regulators of bone resorption (periostin, dentin matrix protein, alkaline phosphatase, collagen 1A1, semaphorin 3A3, metalloproteinase 2, and osteoprotegerin) were higher in the VH group.
Conclusions
Blockage of the RAS system decreases osteoclast differentiation and activity and, consequently, results in decreased strain-induced bone remodeling in orthodontic tooth movement.
Highlights
- •
Many patients take prescription drugs that block the renin-angiotensin system (RAS).
- •
Blockage of the RAS could decrease osteoclast activity.
- •
Drugs that block the RAS might suppress tooth movement and prolong treatment.
- •
AT1 antagonists might provide strategies for modulating tooth movement and bone diseases.
The renin-angiotensin system (RAS) is classically known as a circulating endocrine system regulating blood pressure and electrolyte homeostasis. The main effector peptide in this system is angiotensin II (ANG II), which is formed from angiotensin I by an angiotensin-converting enzyme, a key molecule in this system that interferes in prostaglandin and nitric oxide production. This precursor, angiotensin I, is cleaved from angiotensinogen by renin, a highly selective protease secreted from the juxtaglomerular cells in the kidneys. ANG II exerts its biologic effects through binding to 2 specific angiotensin receptors—angiotensin type 1 receptors (AT1) and angiotensin type 2 receptor (AT2)—that are expressed in many cell types, including osteoclasts and osteoblasts. Drugs that block the RAS, such as losartan (LOS), act by blocking the AT1 receptors and are a main way to control high blood pressure (hypertension), heart failure, kidney failure, and the harmful effects of diabetes.
The relationship between the RAS and bone metabolism is mainly based on the regulation of ANG II on bone metabolism. Different components of the RAS have been found to be synthesized and active in osteoblasts and in osteoclasts, and ANG II has been shown to stimulate bone resorption in osteoblast and osteoclast cocultures. A previous study has also demonstrated expression of the RAS components in osteoblasts and osteoclasts in vivo. Further evidence for a potential role of the RAS in bone metabolism comes from clinical studies. Patients treated with an angiotensin-converting enzyme inhibitor showed increased bone mineral density and reduced fracture risk. Moreover, previous animal studies have shown that excessive activation of RAS causes osteoporosis, mainly by elevation of osteoclastic bone resorption. Along with other factors, ANG II can upregulate the receptor activator of nuclear factor kappa-B ligand (RANKL) expression and downregulate the expression of runt-related transcription factor 2 (RUNX2). These key mediators are responsible for controlling osteoblast and osteoclast differentiation and regulating bone cell activity, leading to reduced bone formation and enhancing bone reabsorption.
As described above, the bone does express components of the RAS, but little is known about the role of the RAS in the context of orthodontic tooth movement (OTM). The aim of this study was to evaluate the effects of blockers of the RAS on osteoclast recruitment and activity using a well-established mouse model of OTM. We hypothesized that the inhibition of the RAS pathway would impair the expression of proinflammatory cytokines, decrease osteoclast recruitment, and consequently reduce OTM.
Material and methods
Forty 10-week-old male C57BL6/J mice were used in this experiment. They were randomly divided into 2 groups: (1) treated with tap water (VH) as the control group, and (2) treated with 10 mg per kilogram per day of LOS (Sigma-Aldrich, St Louis, Mo). The sample size calculation was based on a previous study from our group. No significant weight loss was observed. All treatments followed the ethical regulations of the institutional ethics committee of the Pontifıcal Catholic University of Minas Gerais.
Daily gavages of 100 μL of 10 mg per kilogram per day of LOS or tap water were administered to the LOS and VH groups, respectively. The drug was administered for 6 or 12 days for the histopathologic assays and for 0 or 12 hours for the molecular analyses.
Tooth movement was induced as previously described. Briefly, the mice were anesthetized with 0.2 mL of a xylazine (0.02 mg/mL) and ketamine (50 mg/mL) solution. An orthodontic appliance consisting of a nickel-titanium 0.25 × 0.76-mm coil spring (Lancer Orthodontics, San Marcos, Calif) was bonded by light-cured resin (Transbond; 3M Unitek, Monrovia, Calif) between the maxillary right first molar and both maxillary incisors ( Fig 1 ). The magnitude of force was calibrated by a tension gauge (Shimpo Instruments, Itasca, Ill) to exert a force of 35 g in the mesial direction. No reactivation was performed during the entire experimental period. For the histopathologic analysis, the left side of the maxilla (without an orthodontic appliance) was used as the control.
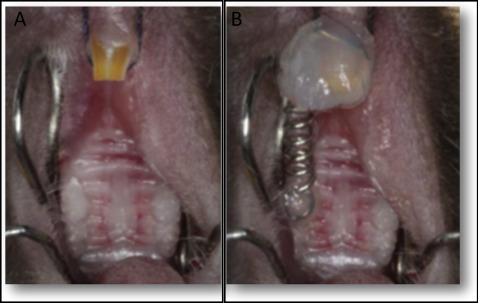
The mice were killed after 6 or 12 days for the histopathologic analysis or after 0 or 12 hours for the molecular analysis. For each set of experiments, 5 animals were used at each time point.
As was previously described, the right and left maxillae halves were fixed in 10% buffered formalin (pH 7.4), decalcified in 14% ethylenediaminetetraacetic acid (pH 7.4) for 20 days, and embedded in paraffin. The samples were cut into sagittal sections 5 μm thick. The sections were stained with tartrate-resistant acid phosphatase (TRAP) (Sigma-Aldrich), counterstained with hematoxylin, and used for the histologic examinations. The mesial periodontal site of the distobuccal root of the first molar was used for osteoclast counts on 5 sections per animal. Osteoclasts were identified as TRAP-positive, multinucleated cells on the bone surface.
Measurement of OTM was performed as previously described. Images of the first and second molars were obtained using an optical microscope (Axioskop 40; Carl Zeiss, Gottingen, Germany) and an adapted digital camera (PowerShot A620; Canon, Tokyo, Japan). ImageJ software (National Institutes of Health) was used to quantify the degree of OTM by measuring the distance between the cementoenamel junction of the first and second molars on the right hemimaxilla in relation to the same measurements for the left hemimaxilla. Five vertical sections per animal were evaluated, and 3 measurements were made for each evaluation; the variability was less than 5%.
RNA extraction and real-time polymerase chain reaction were performed for each animal separately, as previously described. The periodontal ligament and the surrounding alveolar bone were extracted from the maxillary first molars with a stereomicroscope. All gingival tissues, oral mucosa, and teeth were discarded. The periodontal tissues and the alveolar bone that were extracted from the distal area of the distobuccal root of the maxillary first molar were considered the tension site. The mesial area of the same root was considered the compression site. The bone samples (4-8 mg) were frozen in liquid nitrogen after collection and then stored at –80°C. Previous to mRNA extraction, each sample was mechanically fragmented in liquid nitrogen using a bone chisel and homogenized in sterile Milli-Q water with Ultra Turrax (IKA Laboratory Equipment, Staufen, Germany), and subsequently submitted to RNA extraction using TRIZOL reagent (Life Technologies, Carlsbad, Calif), following the manufacturer’s instructions. The mRNA quality and RNA integrity number were assessed with the BioAnalyzer (Agilent, Santa Clara, Calif). The RNA integrity number results ranged between 7.25 and 8.66 (mean, 7.87; SD, 0.36).
Complementary DNA was synthesized using 2 μg of RNA through a reverse transcription reaction (Superscript II; Life Technologies). The targets included molecules known to regulate osteoclast functions: RANK (receptor activator of nuclear factor kappa-B), RANKL, osteoprotegerin, cathepsin K, and metalloproteinase 13. We also analyzed the expression of molecules known to regulate osteoblast functions: periostin, dentin matrix protein, alkaline phosphatase, collagen 1A1, semaphorin 3A3, and metalloproteinase 2. The analyses were performed in ViiA7 equipment (Applied Biosystems, Warrington, United Kingdom) with real-time polymerase chain reaction using TaqMan chemistry (Life Technologies) with inventoried optimized primers/probes sets (Life Technologies) as follows: molecules known to regulate osteoclast functions: RANK (catalog number 433118), RANKL (catalog number 4331182), osteoprotegerin (catalog number 433118), cathepsin K (catalog number 4331182), and metalloproteinase 13 (catalog number 4331182); and molecules known to regulate osteoblast functions: periostin (catalog number 4331182), dentin matrix protein (catalog number 4331182), alkaline phosphatase (catalog number 4331182), collagen 1A1 (catalog number 4331182), semaphorin 3A3 (catalog number 4331182), and metalloproteinase 2 (catalog number 4331182). The basic reaction conditions (40 cycles) were 95°C (10 minutes), 94°C (1 minute), 56°C (1 minute), and 72°C (2 minutes). The mean cycle threshold (Ct) values from duplicate measurements were used to calculate the expression of the target gene, with normalization to an internal control (β-actin) using the 2 −ΔΔCt formula.
Some researchers have used a dosage of antihypertensive drugs that promotes a reduction in blood pressure by 27%. In our study, we chose to use a dose to keep the blood pressure stable to eliminate bias. The mean arterial pressure measurement was evaluated by the tail-cuff method, which is a noninvasive computerized system for measuring blood pressure (Kent Scientific, Torrington, Conn). This tail-cuff blood pressure system uses volume-pressure recording technology to measure the blood pressure in the mouse’s tail. The animals were acclimated to the restraint and tail-cuff inflation for 1 day before the experiments. The restraint platform was maintained at approximately 32°C to 34°C. For each section, the mouse was placed in an acrylic box restraint, and the tail was inserted into a compression cuff that measured the blood pressure 15 times. The average of these values was calculated ( Table ).
Day | Systolic blood pressure (mm Hg) | |
---|---|---|
Vehicle-treated mice | Losartan-treated mice | |
1 day before | 80.7 ± 2.7 | 81.2 ± 1.9 |
1 day after | 77.3 ± 3.1 | 78.3 ± 2.1 |
6 days after | 78.6 ± 2.1 | 79.3 ± 2.6 |
12 days after | 79.8 ± 2.6 | 80.8 ± 2.4 |
Statistical analysis
The data are expressed as the means and standard deviations. The comparisons between the groups were performed using 1-way analysis of variance (ANOVA) followed by the Newman-Keuls multiple comparison test. P ≤0.05 was considered statistically significant.
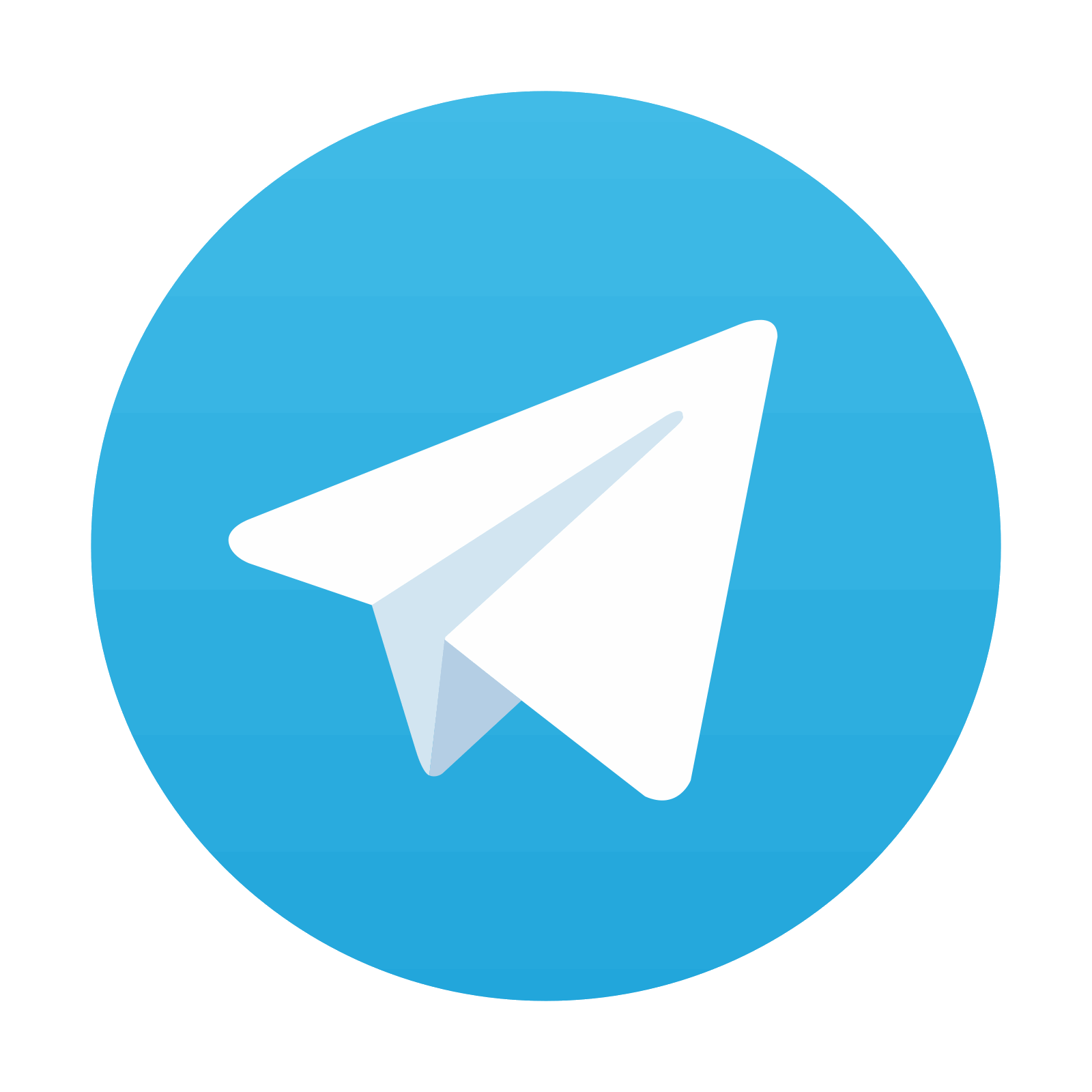
Stay updated, free dental videos. Join our Telegram channel

VIDEdental - Online dental courses
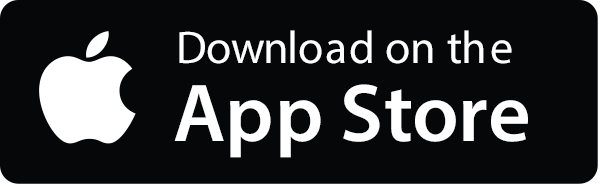
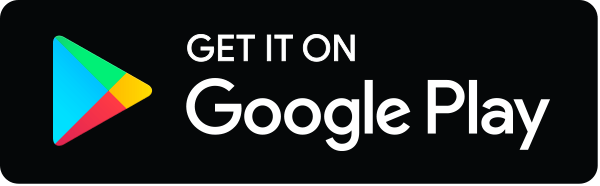