Abstract
Objective
To evaluate the effect of light-curing wavelengths on composite filler particle displacement, and thus to visualize localized polymerization shrinkage in a resin-based composite (RBC) containing camphorquinone (CQ) and Lucirin TPO (TPO).
Methods
Three light-curing units (LCUs) were used to light-cure a RBC containing CQ and TPO: a violet-only, a blue-only, and a dual-wavelength, conventional (Polywave ® , emitting violet and blue wavelengths simultaneously). Zirconia fillers were added to the RBC to act as filler particle displacement tracers. LCUs were characterized for total emitted power (mW) and spectral irradiant output (mW/cm 2 /nm). 2-mm high, 7-mm diameter silanized glass cylindrical specimens were filled in a single increment with the RBC, and micro-computed tomography (μ-CT) scans were obtained before and after light-curing, according to each LCU (n = 6). Filler particle movement identified polymerization shrinkage vectors, traced using software, at five depths (from 0 up to 2 mm): top, top-middle, middle, middle-bottom and bottom.
Results
Considering different RBC depths within the same LCU, use of violet-only and conventional LCUs showed filler particle movement decreased with increased depth. Blue-only LCU showed homogeneous filler particle movement along the depths. Considering the effect of different LCUs within the same depth, filler particle movement within LCUs was not statistically different until the middle of the samples (P > .05). However, at the middle-bottom and bottom depths (1.5 and 2 mm, respectively), blue-only LCU compared to violet-only LCU showed higher magnitude of displacement vector values ( P < .05). Use of the conventional LCU showed filler displacement magnitudes that were not significantly different than blue-only and violet-only LCUs at any depth (P > .05). With respect to the direction of particle movement vectors, use of violet-only LCU showed a greater displacement when close to the incident violet LED; blue-only LCU showed equally distributed particle displacement values within entire depth among the samples; and the conventional LCU showed greater filler displacement closer to the blue LED locations.
Significance
Filler particle displacement in a RBC as a result of light-curing is related to localized application of light wavelength and total emitted power of the light emitted on the top surface of the RBC. When the violet LED is present (violet-only and conventional LCUs), filler particle displacement magnitude decreased with increased depth, while results using the blue-only LED show a more consistent pattern of displacement. Clinically, these results correlate to production of different characteristics of curing within a RBC restoration mass, depending on localized wavelengths applied to the irradiated surface.
1
Introduction
The most commonly used photoinitiator in resin-based composites (RBCs) is camphorquinone (CQ) . This initiator presents its maximum absorption within the blue range of light (around 468–470 nm), and is a bright yellow, colorful photoinitiator, which lessens the potential for esthetic RBC restorations . With the advent of vital tooth bleaching, manufacturers were not able to provide esthetic restorative materials of high enough color value to match those of bleached teeth. This situation led incorporation of alternative, less yellow photoinitiators than CQ , for example, 2,4,6-trimethylbenzoyldiphenylphosphine oxide (Lucirin TPO), which has an absorption range within the short wavelength, visible spectrum (near 410 nm—violet range of light) . However, incorporation of this photoinitiator resulted in two new problems. Firstly, violet wavelength penetrates less deep in RBCs than do blue wavelengths, due to higher attenuation of the lower wavelengths when compared to long ones (resulting in higher decrease in light transmission with increased RBC thickness) and to increased potential for light scattering , thus unlikely effective at deep portions of the restoration . Secondly, light-activated RBCs must not only receive sufficient radiant energy, but also must receive it within the appropriate radiant wavelength range within which the photoinitator system functions. In addition, light emitting diode (LED) light-curing units (LCUs) at that time emitted only blue light, mostly between 445–480 nm. As a result, new LCUs were manufactured that incorporated both blue and violet LEDs, to provide broad-banded radiation (the so-called 3rd generation LED LCUs, also termed multi-wave, multi-peak, or polywave ® LED LCUs) that claim to light-cure all RBCs . These types of LCUs have been shown to promote higher degree of conversion of RBCs containing alternative photoinitiators, when compared to the blue-only LCUs .
With the incorporation of two different colored LEDs, a new issue arose, regarding the spectral distribution of emitted wavelengths at the light guide tip. Studies show wide differences in radiant and spectral emission across the tip ends of some LCUs as well as a lack of uniformity, which could have significant implications on clinical and laboratory results . Thus, use of a single irradiance value to characterize light emission from an LCU is both imprecise and inappropriate , and additional parameters need to be considered when characterizing LCUs, such as emitted power (mW) and spectral irradiant output (mW/cm 2 /nm) . In addition, the photonic requirements of the photoinitiator system of RBCs should be spectrally compatible with the light emitted by the LCU . This information cannot be obtained from a conventional dental curing radiometer .
Current RBCs shrink when light-cured, and the orientation of this shrinkage is fundamental for predicting marginal adaptation and stress distribution . Micro-computed tomography (μ-CT) is a non-destructive, 3D, high-resolution imaging technique effective in evaluating volumetric polymerization shrinkage , cementation film thickness , and polymerization shrinkage vectors , among others. Shrinkage vectors can be used to analyze the extent and direction in which polymerization shrinkage occurs, and represents movement of RBCs when the material is light-cured . Also, it is suggested that shrinkage vectors can be correlated to degree of conversion, hardness, and bonding failures within a RBC restoration, because bonding failures found in the restoration floor might occur as a result of lower copolymerization of monomers, because of insufficient delivery of light energy at this location .
To track shrinkage vectors inside the RBC mass, different techniques have been utilized, such as tracking air bubbles , fluorescent fillers , glass fillers , zirconia fillers , as well as finite element analysis . However, shrinkage vectors showed to be non-independent parameters; their direction is controlled by external factors such as boundary adhesion conditions, shape of the preparation, and characteristics of the boundary margins (enamel/dentin) . Also, the magnitude of shrinkage vectors depends on the chemical composition of the RBC, its photoinitiator type and degree of conversion, which are also affected by the intensity of the light source applied, its depth of penetration, and the exposure duration used .
Wavelength-dependent photosensitivity of commercial RBCs is related to the photoinitiators within their composition, the types and amounts of which are not usually listed. However, these factors vary greatly among different brands of similar shades, or even among different shades within the same product brand . Moreover, a better curing at the top surface of RBCs when compared to the bottom surface has been shown .
The non-homogeneity of emitted power and wavelength distribution of LCUs can greatly affect the performance of a restoration in mouth . Clinically, simply by changing the position of the LCU can result in a dramatic effect on the properties of a restoration at different locations .
Thus, the purpose of this study was to characterize the effect of application of different emitted powers and wavelength emissions from experimental LCUs (one emitting violet-only, one blue-only, and a conventional, commercial product emitting both violet and blue wavelengths simultaneously) on vector-based movement of radiopaque filler particles inside a RBC mass containing both CQ and TPO as photoinitiators. The hypotheses tested were that (1) the magnitude of filler particle movement (polymerization shrinkage) beneath either the violet or blue LED would be similar at the top surface, but (2) as RBC depth increased, filler particle movement, and thus polymerization shrinkage, would become lower for RBC underneath the violet light than for blue light, where lower filler movement is correlated to less shrinkage and thus, less curing. In addition, (3) when both colors of LED were activated simultaneously (conventional LCU), a synergistic effect would be noted.
2
Materials and methods
Eighteen cylindrical glass matrices specially prepared for this study (7 mm inner diameter × 2 mm depth × 13 mm outside diameter) were obtained by serially cutting a cylindrical glass tube (MPL, Piracicaba, SP, Brazil), glued (Super Bonder, Loctite, SP, Brazil) to microscope slides. The outside walls of each tube were painted with black nail varnish (Colorama, SP, Brazil) to prevent light dissipation through the matrix walls. After that, the prepared cylinders were randomly divided into 3 groups (n = 6), with respect to the LCU used. The LCUs used were all based on a commercially available Polywave ® LED unit (Bluephase 20i, Ivoclar Vivadent, Schaan, Liechtenstein). The manufacturer supplied one LCU, having both colors of LEDs activated when the unit was turned on (conventional); one LCU where only the violet LED was activated when the unit was turned on (violet-only); and one LCU where only the blue LEDs in the chip array were activated when the light was turned on (blue-only).
The internal surfaces (sides and bottom) of the glass cylindrical matrices were coated with a silane agent (Monobond Plus, Ivoclar Vivadent) to ensure RBC adherence to the glass boundary, and identification marks, according to the different LEDs from the LCUs, were made on the matrices, in order to identify the exact locations where the different LEDs were irradiating. A single RBC was custom-formulated from a commercialized composite (Empress Direct Trans 30, Ivoclar Vivadent), which was blended by the manufacturer, and contained different photoinitiators (CQ and TPO) as well as specialized, spherical, radiopaque zirconia fillers that were homogeneously incorporated and functioned as radiopaque tracers. The special zirconia fillers, 2.5 wt%, 50 μm diameter (Tosoh Corporation, Tokyo, Japan), were similar to those used in previous research , and permitted radiographic identification of their movement, from which polymerization shrinkage vectors (magnitude and direction) were calculated, as a result of positional changes resulting from light-curing. The composition of the materials is listed on Table 1 .
Material | Composition | Manufacturer |
---|---|---|
Model composite: Empress Direct T30 shade with zirconia oxide fillers | 22.5 wt% monomer; 10.0 wt% mixed oxide; 59.0 wt% Ba–glass filler; 6.0 wt% prepolymerized filler; 2.5 wt% zirconia; photoinitiators | Ivoclar Vivadent, Schaan, Liechtenstein |
Silane bonding agent: Monobond Plus | Ethanol; 3-trimethoxysilylpropyl methacrylate; methacrylated phosphoric acid ester | |
Zirconia fillers | Zirconia nanofiller 50 μm diameter | Tosoh Corporation, Tokyo, Japan |
Single violet peak LED (violet-only experimental Bluephase 20i) | Violet-only LED LCU | Ivoclar Vivadent, Schaan, Liechtenstein |
Single blue peak LED (blue-only experimental Bluephase 20i) | Blue-only LED LCU | |
LED polywave blue/violet (Bluephase 20i) | Conventional LED LCU |
2.1
Light-curing unit characterizations
Various aspects of the LCUs were taken into consideration for this testing ( Fig. 1 ). To characterize radiant emission from each LCU, emitted power was measured using a calibrated, NIST-traceable thermopile (Fieldmate, Coherent Inc., USA), using a PM-10 detector. After that, a measure of the spectral irradiant profile was performed, using a 6” integrating sphere (USS 060 SF, Labsphere Inc., Sutton, NH, USA) containing a NIST-traceable light source, attached to a spectroradiometer (USB2000, Ocean Optics, Dunedin, FL, USA), which provides output to computer software (Spectra Suite v5.1, Ocean Optics Inc., Dunedin, FL, USA).
Distribution of the emitted power over the surface of the light tip was measured using a modified laser beam-profiling instrument (Model LBA-FW-SCOR20, Spiricon Inc., Logan, UT, USA). The emitting end of the light tip was imaged directly by the camera. To isolate locations of different wavelengths in the tip end, narrow bandpass filters (10 nm) were placed between the camera lens and light tip: 410 nm and 460 nm (Edmund Scientific, Redbank, NJ, USA). The emitted power (mW) values were measured 3 times using the thermopile as well as the integrating sphere, averaged, and entered into the beam analysis software (LBA-FW for Windows XP and Vista, V 4.89, Spiricon, Inc., Logan, UT, USA). By doing so, the response of each camera pixel was calibrated, such that the total of all imaged pixels equaled the measured light power output. Software then created a colorized pattern of the power level distribution levels over the tip end of each LCU. Specific attention was taken in order to identify and map those locations on the light tip end where the individual wavelength emissions were produced, so that differences in tracer filler movement could be correlated to the specific locations of both localized power values and spectral variations.
2.2
Shrinkage vectors
The cylindrical dark glass matrices were filled with the RBC in a single increment. A high-resolution micro-computed tomography instrument (μ-CT) (Bruker Skyscan 1172, Bruker, Kontich, Belgium) was used to scan the RBC material, before and after light-curing. LCUs were also marked according to the location of each LED, so the marks from glass matrices and from the LCUs could be correlated and maintained at the same position when light-curing was performed, enabling the vectors analysis. Operating conditions for the μ-CT were as follows: 100 kV voltage and 100 μA current, and 9.7 μm pixel size. Specimens were covered with a radiolucent dark cap, to avoid RBC curing during the initial μ-CT scan (before light-curing), while the baseline scans were obtained. After the first scan, the dark cap was removed and, outside of the μ-CT device, the specimens were light-cured for 30 s, using the designed LCU at a standardized, fixed distance of 2 mm from the sample surface, after which, the specimen was relocated in the μ-CT device for the second scan (after light-curing). Special attention was given to marking the orientation of the LCU tip with the specimen, to ensure identification of location of the emitting LEDs with respect to the exposed RBC surface.
The data from before and after light-curing were imported into open source software (3D Slicer—Version 4.6, https://www.slicer.org/ ) on a Windows-based workstation, and images from before and after light-curing were perfectly aligned and superimposed using the marks previously made on the glass matrices; the tolerance for the registration was set to 0.0001 mm. From each sample, serial 2D images were put together to form 3D images. Eighty-five given points from each sample were selected according to depth and position within the diameter of the sample, and the same points were evaluated among the samples ( Fig. 2 ). Composite depth locations were defined as follows: top (0 mm depth), top-middle (0.5 mm deep), middle (1 mm deep), middle-bottom (1.5 mm deep) and bottom (2 mm deep). Each data point was identified and paired between each of the 2 scans, in order to trace the filler particle movement. Movement distance of each filler particle after light-curing was then obtained with respect to its vector-based directions (x, y, and z axes).
2.3
Statistical analyses
Data normality was checked using the Shapiro–Wilk test ( P = .979), with respect to the different positions within the samples (top-bottom). Vector-based particle movement length among LCUs and LED location positions were analyzed using a 2-way, Split-Plot ANOVA. When the effects were statistically significant, post-hoc analyses were performed using the Fisher LSD procedure. Statistical analyses were performed using software (SPSS, V22, IBM Corp., Armonk, NY). The level of significance was indicated by a type 1 error rate less than 5% ( α = .05).
2
Materials and methods
Eighteen cylindrical glass matrices specially prepared for this study (7 mm inner diameter × 2 mm depth × 13 mm outside diameter) were obtained by serially cutting a cylindrical glass tube (MPL, Piracicaba, SP, Brazil), glued (Super Bonder, Loctite, SP, Brazil) to microscope slides. The outside walls of each tube were painted with black nail varnish (Colorama, SP, Brazil) to prevent light dissipation through the matrix walls. After that, the prepared cylinders were randomly divided into 3 groups (n = 6), with respect to the LCU used. The LCUs used were all based on a commercially available Polywave ® LED unit (Bluephase 20i, Ivoclar Vivadent, Schaan, Liechtenstein). The manufacturer supplied one LCU, having both colors of LEDs activated when the unit was turned on (conventional); one LCU where only the violet LED was activated when the unit was turned on (violet-only); and one LCU where only the blue LEDs in the chip array were activated when the light was turned on (blue-only).
The internal surfaces (sides and bottom) of the glass cylindrical matrices were coated with a silane agent (Monobond Plus, Ivoclar Vivadent) to ensure RBC adherence to the glass boundary, and identification marks, according to the different LEDs from the LCUs, were made on the matrices, in order to identify the exact locations where the different LEDs were irradiating. A single RBC was custom-formulated from a commercialized composite (Empress Direct Trans 30, Ivoclar Vivadent), which was blended by the manufacturer, and contained different photoinitiators (CQ and TPO) as well as specialized, spherical, radiopaque zirconia fillers that were homogeneously incorporated and functioned as radiopaque tracers. The special zirconia fillers, 2.5 wt%, 50 μm diameter (Tosoh Corporation, Tokyo, Japan), were similar to those used in previous research , and permitted radiographic identification of their movement, from which polymerization shrinkage vectors (magnitude and direction) were calculated, as a result of positional changes resulting from light-curing. The composition of the materials is listed on Table 1 .
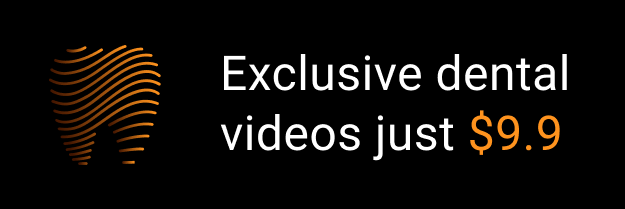