Abstract
Objectives
The purpose of the present study was to investigate the effect of ball milling on the initial mercury vapor release rate and mechanical properties such as compressive strength, diametral tensile strength and creep value, of the dispersed-type dental amalgam, and comparison was made with respect to two commercial amalgam alloys.
Methods
Ball milling was employed to modify the configuration of the originally spherical-shaped Ag–Cu–Pd dispersant alloy particles. Improvement in mechanical properties while maintaining a low early-stage mercury vapor release rate of the amalgam is attempted.
Results
The experimental results show that the amalgam (AmB10) which was made from Ag–Cu–Pd dispersant alloy particles that were ball-milled for 10 min and heat-treated at 300 °C for 2 days exhibited a low initial mercury vapor release rate of 69 pg/mm 2 /s, which was comparable with that of commercial amalgam alloy Tytin (68 pg/mm 2 /s), and was lower than that of Dispersalloy (73 pg/mm 2 /s). As for mechanical properties, amalgam AmB10 exhibited the highest 1 h compressive strength (228 MPa), which was higher than that of commercial amalgam alloy Dispersalloy by 72%; while its 24 h diametral tensile strength was also the highest (177 MPa), and was higher than that of Dispersalloy by 55%. Furthermore, the creep value of the amalgams made from Ag–Cu–Pd alloy particles with 10 min ball-milling and heat treatment at 300 °C for 2 days was measured to be 0.12%, which was about 20% that of Dispersalloy.
Significance
It is found that ball milling of the dispersant Ag–Cu–Pd alloy particles for 10 min was able to modify the configuration of the alloy particles into irregular-shapes. Subsequently, heat treatment at 300 °C significantly lowered the initial mercury vapor release rate, increased its 1 h compressive strength and 1 h diametral tensile strength, and lowered its creep value.
1
Introduction
Dental amalgam has been the most widely used tooth filling material for decades due to its high strength, durability and wear resistance , as well as long-term satisfactory clinical performance . Nevertheless, there is an increasing interest in the study of potential mercury hazard from dental amalgam. Mercury vapor released from newly placed and aged dental amalgams, including low-copper and high-copper amalgams, has been identified . The effects of mercury on human health as well as on environmental pollution remain a major concern among both the scientific community and the general public.
Since the emergence of dispersed-type palladium (Pd) amalgams in the mid-1980s, a number of promising results from Pd dispersants and Pd dispersant amalgams have been reported. The Pd-induced improvements include superior corrosion resistance , higher compressive strength , lower creep , inhibition of the formation of Cu 6 Sn 5 phase and the transformation of Ag 2 Hg 3 (γ1) to Ag–Hg (β1) at elevated temperatures, as well as drastically reduced early stage mercury vapor release from the matrix γ1 phase . It was also reported that the addition of Pd in an Ag–25Sn–12Cu amalgam alloy decreased the amount of released mercury vapor during setting . Amalgams containing Pd also showed improved surface luster and less electrochemical activity .
It was reported that the composition, particle size and shape of amalgam alloys could all affect the handleability, phase contents and properties of amalgam . Compared to those from lathe-cut irregular-shaped particles, amalgams fabricated from spherical-shaped (atomized) particles generally exhibited shorter working time , less mercury content , less sensitivity to condensation pressure , less dimensional change , lower creep and higher compressive strength . Disadvantages of amalgams prepared from spherical particles include their relatively high mercury vapor release rates and the reported more extensive microleakage than that from lathe-cut irregular-shaped alloy particles .
Process conditions are also critical to the performance of dental amalgam. Among many process details, the relief of the process-induced internal stresses from amalgam alloy particles is perhaps the most critical issue. Particularly during manufacturing of irregular-type amalgam particles, the cutting or milling-induced stresses in the particles can cause changes in amalgam alloy properties, such as amalgamation rate and dimensional changes during hardening .
Generally the process-induced residual stresses can be relieved by carrying out an annealing treatment at a moderate temperature on the alloy. It was reported that, when milled amalgam alloy was maintained at room temperature, its internal residual stress was relieved over a period of weeks or months . When annealed at 100 °C, this stress-relieving process could be shortened to several hours . Higher annealing temperatures (up to 240 °C) under inert gas atmosphere (N 2 /H 2 = 80/20) were also disclosed in the US patent No. 4859412 . In a microstructural study of Ag–Cu–Pd alloy dispersant particles, Chern Lin et al. indicated that the lathe cutting-induced residual stress in the particles, which was accompanied with a phenomenon of X-ray diffraction (XRD) peak broadening, could be largely removed by annealing the alloy particles in argon atmosphere at 90 °C for at least 1 week.
The purpose of this study was to investigate the effect of ball milling on the initial mercury vapor release rate and mechanical properties, such as compressive strength, diametral tensile strength and creep, of amalgams, and comparison was made with respect to commercial amalgam alloys. Improvement in mechanical properties while maintaining a low early-stage mercury vapor release rate of the amalgam is attempted.
2
Materials and methods
Spherical-shaped particles of matrix alloy Ag–Cu–Sn (70 wt% Ag–4 wt% Cu–26 wt% Sn) and dispersant alloy Ag–Cu–Pd (62 wt% Ag–28 wt% Cu–10 wt% Pd) were fabricated using a commercial atomizing system (HTJ Co., New York, USA). The majority of these atomized spherical particles are less than 45 μm in diameter. Ball-milling was carried out on spherical Ag–Cu–Pd alloy particles by using a SPEX-8000 ball mill (A.O. Smith Corp, USA) containing twenty stainless steel balls, including four of 1/2 in. in diameter, six of 3/8 in. in diameter, and 10 of 1/4 in. in diameter.
The spherical-shaped Ag–Cu–Pd alloy particles were subjected to four different duration of time of ball milling, i.e., 0, 5, 10, and 60 min. After ball milling, the Ag–Cu–Pd alloy particles were sieved through ASTM specification #325 (<45 μm) sieves. The sieved-through alloy particles were contained in glass vials, which were first subjected to vacuum and then filled with argon gas and then were sealed off with a torch. These glass vials were then heated to 300 °C with a heating rate of 5 °C/min, and kept at 300 °C for 2 days. After the heat treatment, the ball-milled alloy particles were cleaned with 10% HCl solution to remove the naturally occurring surface oxide of the alloy particles. The sample coding and process conditions for the above Ag–Cu–Pd alloy particles are summarized in Table 1 .
Ag–Cu–Pd alloy | Ball-milling time (min) | Heat treatment temperature and time |
---|---|---|
B0H0 | None | None |
B0 | None | 300 °C, 2 days |
B5 | 5 | 300 °C, 2 days |
B10 | 10 | 300 °C, 2 days |
B60 | 60 | 300 °C, 2 days |
Trituration of alloy/mercury mixture was carried out in a closed capsule using a commercial triturator (Crescent LP-60, Lyons, IL, USA). The alloy contained two parts of Ag–Cu–Sn powder and one part of Ag–Cu–Pd powder (by weight). As a result, the alloy had an overall composition of 67.2 wt% Ag, 12.0 wt% Cu, 17.4 wt% Sn, and 3.4 wt% Pd. The triturated amalgam mass was hand-condensed in a disk-shaped acrylic die of 10 mm in diameter and 2 mm in depth for phase identification and mercury vapor release measurement. Table 2 shows the codes of different amalgam alloy samples and corresponding process conditions of each alloy particles.
Codes | Ag–Cu–Pd | Ag–Cu–Sn | |
---|---|---|---|
Ball-milling time (min) | Heat-treatment conditions | Heat-treatment conditions | |
AmB0H3 | 0 | 300 °C, 2 days | 100 °C, 2 days |
AmB5H3 | 5 | 300 °C, 2 days | 100 °C, 2 days |
AmB10H3 | 10 | 300 °C, 2 days | 100 °C, 2 days |
AmB60H3 | 60 | 300 °C, 2 days | 100 °C, 2 days |
Phase identification of the series of amalgams was conducted using a Rigaku D-Max/IIB X-ray diffractometer (Rigaku Crop., Tokyo, Japan) operated at 30 kV and 20 mA. Cu Kα radiation scanning from 30° to 70° (2 ) was used at a scan speed of 1°/min. The microstructure and chemical composition of the amalgams were characterized using scanning electron microscope (SEM) equipped with an X-ray energy dispersive spectrometer (EDS, Voyager II, Japan). Field emission scanning electron microscope (FE-SEM, XL 40, PHILIPS, Holland), and high resolution scanning electron microscope (Hitachi S-4100 Field Emission SEM, Hitachi, Japan) were used in this study.
Cylindrical-shaped specimens (4 mm in diameter and 8 mm in height) were used for compressive strength, diametral tensile strength (DTS) and creep tests. The specification of the specimens was complied with ADA spec. No. 1 for the measurement of mechanical properties of dental amalgams. The compressive strength and DTS of the amalgams were measured using a SHIMADZU AUTOGRAPH AGS-500D dynamic mechanical tester (Shimadzu Corp., Kyoto, Japan) at a cross-head speed of 0.5 mm/min. The compressive strength of each specimen was calculated according to the equation, P = 4 F / πD 2 , where P is compressive strength in MPa, F the fracture load in N, and D the diameter of the specimen in mm. The DTS value was calculated according to the equation, σ t = 2 F / πDt , where σ t is DTS in MPa, F the fracture load in N, D the diameter of the specimen in mm, and t the specimen thickness in mm. Before creep measurement, the amalgams were aged at 37 °C for 7 days. The creep value of amalgam at 37 °C under a uniaxial compression load of 36 MPa was calculated using the equation, creep value = (Δ L 4 − Δ L 1 )/ L 0 × 100%, where L 0 is the original length of the specimens, Δ L 1 the creep strain after 1 h, and Δ L 4 the creep strain after 4 h (Δ L 1 = L 1 − L 0 , Δ L 4 = L 4 − L 0 ). All compressive strength, DTS and creep value are averages of five tests under each condition. One-way ANOVA was used to evaluate data and Scheffé multiple comparison test was run to determine the significance of deviations among each group. In all cases the statistical difference was considered significant at P < 0.05 level.
The initial mercury vapor release rate was measured with a Jerome gold film mercury vapor analyzer (JEROME 431-X, Arizona Instrument LLC Phoenix, AZ, USA). Atmospheric-pressure air was blown continuously through the tubing of an aquarium air pump at a rate of 750 mL/min which is equal to the air-suction rate of the mercury vapor analyzer. The first reading was taken at 90 s after trituration. After each measurement, the specimen was removed from the detection chamber and clean air was pumped through the analyzer until the reading dropped back to zero to ensure that no mercury vapor was left in the chamber .
2
Materials and methods
Spherical-shaped particles of matrix alloy Ag–Cu–Sn (70 wt% Ag–4 wt% Cu–26 wt% Sn) and dispersant alloy Ag–Cu–Pd (62 wt% Ag–28 wt% Cu–10 wt% Pd) were fabricated using a commercial atomizing system (HTJ Co., New York, USA). The majority of these atomized spherical particles are less than 45 μm in diameter. Ball-milling was carried out on spherical Ag–Cu–Pd alloy particles by using a SPEX-8000 ball mill (A.O. Smith Corp, USA) containing twenty stainless steel balls, including four of 1/2 in. in diameter, six of 3/8 in. in diameter, and 10 of 1/4 in. in diameter.
The spherical-shaped Ag–Cu–Pd alloy particles were subjected to four different duration of time of ball milling, i.e., 0, 5, 10, and 60 min. After ball milling, the Ag–Cu–Pd alloy particles were sieved through ASTM specification #325 (<45 μm) sieves. The sieved-through alloy particles were contained in glass vials, which were first subjected to vacuum and then filled with argon gas and then were sealed off with a torch. These glass vials were then heated to 300 °C with a heating rate of 5 °C/min, and kept at 300 °C for 2 days. After the heat treatment, the ball-milled alloy particles were cleaned with 10% HCl solution to remove the naturally occurring surface oxide of the alloy particles. The sample coding and process conditions for the above Ag–Cu–Pd alloy particles are summarized in Table 1 .
Ag–Cu–Pd alloy | Ball-milling time (min) | Heat treatment temperature and time |
---|---|---|
B0H0 | None | None |
B0 | None | 300 °C, 2 days |
B5 | 5 | 300 °C, 2 days |
B10 | 10 | 300 °C, 2 days |
B60 | 60 | 300 °C, 2 days |
Trituration of alloy/mercury mixture was carried out in a closed capsule using a commercial triturator (Crescent LP-60, Lyons, IL, USA). The alloy contained two parts of Ag–Cu–Sn powder and one part of Ag–Cu–Pd powder (by weight). As a result, the alloy had an overall composition of 67.2 wt% Ag, 12.0 wt% Cu, 17.4 wt% Sn, and 3.4 wt% Pd. The triturated amalgam mass was hand-condensed in a disk-shaped acrylic die of 10 mm in diameter and 2 mm in depth for phase identification and mercury vapor release measurement. Table 2 shows the codes of different amalgam alloy samples and corresponding process conditions of each alloy particles.
Codes | Ag–Cu–Pd | Ag–Cu–Sn | |
---|---|---|---|
Ball-milling time (min) | Heat-treatment conditions | Heat-treatment conditions | |
AmB0H3 | 0 | 300 °C, 2 days | 100 °C, 2 days |
AmB5H3 | 5 | 300 °C, 2 days | 100 °C, 2 days |
AmB10H3 | 10 | 300 °C, 2 days | 100 °C, 2 days |
AmB60H3 | 60 | 300 °C, 2 days | 100 °C, 2 days |
Phase identification of the series of amalgams was conducted using a Rigaku D-Max/IIB X-ray diffractometer (Rigaku Crop., Tokyo, Japan) operated at 30 kV and 20 mA. Cu Kα radiation scanning from 30° to 70° (2 ) was used at a scan speed of 1°/min. The microstructure and chemical composition of the amalgams were characterized using scanning electron microscope (SEM) equipped with an X-ray energy dispersive spectrometer (EDS, Voyager II, Japan). Field emission scanning electron microscope (FE-SEM, XL 40, PHILIPS, Holland), and high resolution scanning electron microscope (Hitachi S-4100 Field Emission SEM, Hitachi, Japan) were used in this study.
Cylindrical-shaped specimens (4 mm in diameter and 8 mm in height) were used for compressive strength, diametral tensile strength (DTS) and creep tests. The specification of the specimens was complied with ADA spec. No. 1 for the measurement of mechanical properties of dental amalgams. The compressive strength and DTS of the amalgams were measured using a SHIMADZU AUTOGRAPH AGS-500D dynamic mechanical tester (Shimadzu Corp., Kyoto, Japan) at a cross-head speed of 0.5 mm/min. The compressive strength of each specimen was calculated according to the equation, P = 4 F / πD 2 , where P is compressive strength in MPa, F the fracture load in N, and D the diameter of the specimen in mm. The DTS value was calculated according to the equation, σ t = 2 F / πDt , where σ t is DTS in MPa, F the fracture load in N, D the diameter of the specimen in mm, and t the specimen thickness in mm. Before creep measurement, the amalgams were aged at 37 °C for 7 days. The creep value of amalgam at 37 °C under a uniaxial compression load of 36 MPa was calculated using the equation, creep value = (Δ L 4 − Δ L 1 )/ L 0 × 100%, where L 0 is the original length of the specimens, Δ L 1 the creep strain after 1 h, and Δ L 4 the creep strain after 4 h (Δ L 1 = L 1 − L 0 , Δ L 4 = L 4 − L 0 ). All compressive strength, DTS and creep value are averages of five tests under each condition. One-way ANOVA was used to evaluate data and Scheffé multiple comparison test was run to determine the significance of deviations among each group. In all cases the statistical difference was considered significant at P < 0.05 level.
The initial mercury vapor release rate was measured with a Jerome gold film mercury vapor analyzer (JEROME 431-X, Arizona Instrument LLC Phoenix, AZ, USA). Atmospheric-pressure air was blown continuously through the tubing of an aquarium air pump at a rate of 750 mL/min which is equal to the air-suction rate of the mercury vapor analyzer. The first reading was taken at 90 s after trituration. After each measurement, the specimen was removed from the detection chamber and clean air was pumped through the analyzer until the reading dropped back to zero to ensure that no mercury vapor was left in the chamber .
3
Results and discussion
3.1
XRD analysis of Ag–Cu–Pd alloy particles with different ball-milling time
Fig. 1 shows the X-ray diffraction (XRD) patterns of Ag–Cu–Pd alloy particles. It is observed that in B0H0 (spray-atomized alloy particles, without heat treatment nor ball milling) the Ag, Cu, Cu 3 Pd peaks were broadened due to the residual stress in the particles caused by rapid solidification during spray atomization process, whereas in B0 (heat treated at 300 °C for 2 days but without ball milling) sharp diffraction peaks of Ag, Cu and Cu 3 Pd appeared, indicating that the milling-induced residual stress had been largely relaxed by the heat treatment. It appears that in B5 the diffraction peaks were slightly diminished by a 5 min of ball milling with respect to B0, that further milling of 10 min as in B10 the diffraction peak intensities increased, and then that the diffraction peaks decreased at B60. Among the ball-milled particles, B10 exhibited the strongest diffraction peaks, indicating the best crystallinity among these samples.

3.2
SEM observation of microstructures of Ag–Cu–Pd alloy particles with different ball-milling time
Ag–Cu–Pd dispersant particles were ball-milled for 0, 5, 10, and 60 min, each followed by heat treatment at 300 °C for 2 days. Fig. 2 shows the SEM images of the ball-milled Ag–Cu–Pd particles, and dark precipitates can be seen in the backscattered electron (BSE) images at 5000× magnification. BSE images at 15,000× magnification further reveal the shape of the dark precipitates in both B0 and B10 alloy particles. Fig. 3 shows that the dark precipitates are more uniformly distributed in the ball-milled Ag–Cu–Pd particles B10 than those in the non ball-milled B0. This more uniform distribution of dark precipitates may be attributed to ball milling, by which the Ag–Cu–Pd particles were repeatedly plastically deformed, thereby providing more uniformly distributed nucleation sites for to dark precipitates to form during subsequent heat treatment.
3.3
Hg/alloy ratio, working time and setting time
For dental use, the amalgam is typically prepared by trituration of mercury by weight from 0.8:1 to 1.5:1 ratio with alloy powders, while the most suitable range from 0.9:1 to 1.4:1, with 1:1 being the optimum ratio . Table 3 shows the Hg/alloy ratio for amalgamation, working time and setting time of various samples AmB0, AmB5, AmB10, AmB60, which were all prepared with an Hg/alloy ratio of 1.4:1. In this series of samples, AmB0 had the longest working time (6 min), AmB60 the shortest (2.5 min). Similarly, AmB0 had the longest setting time (10 min), while AmB60 the shortest (4 min). It is observed that both the working time and the setting time decreased as the ball milling time increased.
Sample | Hg/alloy ratio | Working time (min) | Setting time (min) |
---|---|---|---|
AmB0 | 1.4 | 6 | 10 |
AmB5 | 1.4 | 4.5 | 8 |
AmB10 | 1.4 | 3 | 5 |
AmB60 | 1.4 | 2.5 | 4 |
Dispersalloy | 0.99 | 7 | 12 |
Tytin | 0.74 | 10 | 17 |
3.4
Microstructures
3.4.1
X-ray diffraction
The XRD results are shown in Fig. 4 (a)–(d), where main diffraction peaks of γ 1 (Ag 2 Hg 3 ), ε (Cu 3 Sn), η′ (Cu 6 Sn 5 ) and unreacted γ (Ag 3 Sn) were identified. It is interesting to note that γ 2 phase was not detected in all of the four process conditions. It is known that γ 2 is the weakest phase in amalgam in terms of mechanical properties, such as compressive strength, DTS and creep value, and it also contributes to the reduction of amalgam’s corrosion resistance. Chen et al. reported that the γ 2 phase was observed only in the amalgams fabricated from irregular-shaped dispersant particles; moreover, after aging for 7 days, γ 2 phase was not detectable in these amalgams.
XRD Intensities and intensity ratios (to γ 1 (2 2 2)) of these peaks in the various amalgams are shown in Fig. 5 . Intensity ratios were taken in order to eliminate day-to-day instrument variations of XRD intensity measurement. The highest intensity peak of γ 1 phase (3 0 0) was not used for comparison due to its overlapping with other peaks such as Ag (1 1 1) and γ 1 (4 1 1). The use of XRD peak ratio with respect to γ 1 (2 2 2) intensity was reported by Marshall and Marshall .
The effect of ball milling on XRD intensity was evident in the γ 1 (2 2 2) phase and the η′ (1 0 1) phase. It is observed in Fig. 5 (a) and (b) that the γ 1 (2 2 2) and the η′ (1 0 1) phase intensities increased markedly for all the ball-milled amalgams (AmB5, AmB10 and AmB60) with respect to their corresponding non ball-milled amalgam (AmB0), indicating that ball milling greatly enhanced the amalgamation reaction rates, mostly likely due to the increased particle surface area for amalgamation due to ball milling.
Fig. 5 (c) shows that γ (0 1 2) peak intensity decreases as aging time increases. However, γ (0 1 2) peak was not detected after aging for 7 days, indicating that the initially unreacted γ phase of the amalgam was mostly consumed after aging for 7 days. Fig. 5 (d) shows that ball milling had certain impact on the formation of η′ phase, as can be seen that the η′/γ 1 ratios of ball-milled amalgams (AmB5, AmB10, and AmB60) are generally lower than that of the non ball-milled amalgam (AmB0). In addition, it is also noticed that on average the η′/γ 1 ratios of ball-milled amalgams decreases as the ball milling time was increased, indicating that prolonged ball milling might suppress the formation of η′ phase during amalgamation.
As shown in Fig. 5 (e), the γ (0 1 2)/γ 1 (2 2 2) ratio of the amalgams (AmB5, AmB10, and AmB60) prepared from ball-milled Ag–Cu–Pd alloy particles were approximately 50% lower than that which was without ball milling (AmB0). It is also noted in Fig. 5 (e) that the γ/γ 1 ratios of 7 days aging all came down to zero for all of the three ball-milled amalgams, indicating that the amalgamation reaction proceeded at a much faster rate for ball-milled amalgams than did the non ball-milled amalgam, and the originally unreacted γ phase was probably all but consumed after 24 h of aging. This might be explained by the particle size reduction of Ag–Cu–Pd dispersant particles by ball milling, which increased the amalgamation rate and consequently assisted in the completion of amalgamation, thereby the disappearance of the γ (Ag 3 Sn) phase. Since the Ag–Cu–Pd and Ag–Cu–Sn alloy particles in this study were all subjected to heat treatment for 2 days at 300 °C and 100 °C (see Table 2 ), respectively, after ball milling, the stresses that were introduced by various time of ball milling are considered to have been annealed out by the heat treatments.
3.4.2
SEM observations of microstructures
Fig. 6 shows both the secondary electron (SE) and the backscattered electron (BSE) images of four amalgams, namely, AmB0, AmB5, AmB10, and AmB60. For AmB0 Fig. 6 (a) and (b), the dark round particles of different sizes are Ag–Cu–Sn dispersant alloy particles, and it is observed that only a thin layer at the outer region of Ag–Cu–Pd dispersant particles was reacted, while all the interior body of the dispersants remain un-reacted. Throughout the Ag–Cu–Sn alloy particles, they appear to have all been reacted but only a thin dark periphery, consisting most likely of η′ Cu–Sn crystals, remained around the particles. The inner region of the reacted Ag–Cu–Sn particles show the same shade of gray as that of the γ phase of the matrix, indicating Hg was able to diffuse into the core of the particles to form the gray-appearing γ phase.
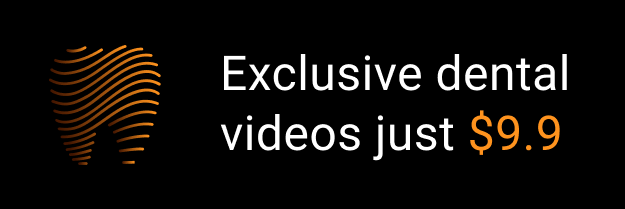