Abstract
The objective of this study was to investigate the influence of maxillary alveolar bone on the stress distribution of zygomatic implants. A three-dimensional finite element model was created of half of a skull. Two zygomatic implants were modelled, placed in the skull supported by the zygomatic bone and the maxillary alveolar bone and connected by a fixed bridge. This model was duplicated, and the area of the maxillary alveolar bone supporting the implants was removed. Occlusal and lateral forces were applied to both models and the maximum von Mises stresses were recorded. Higher maximum stresses were noted in the model with no alveolar support. Occlusal stresses were higher than lateral stresses in the model with no alveolar support. Low stresses were noted in the zygomatic bone in both models. In conclusion, maxillary alveolar bone support is beneficial in the distribution of forces for zygomatic implants.
Zygomatic implants are used in the severely atrophic maxilla to support fixed or removable prosthodontics. Intra and extra-sinus positions for zygomatic implants have been described, both with high success rates. These provide an alternative for patients who do not have sufficient maxillary bone to retain conventional dental implants and for whom grafting procedures are unsuitable.
Brånemark originally proposed that the zygomatic implant should be placed, via the sinus, into the zygomatic bone with support from the maxillary alveolar or palatal bone coronally. This, along with cross arch stabilization from other conventional and zygomatic implants provides a sound foundation for a fixed arch bridge. Since then, new protocols have been developed, showing similar success rates for bridges supported only by zygomatic implants and in some cases immediately loaded. Malo et al. reported on a case series of zygomatic implants placed without any support from the maxillary alveolar or palatal bone. Zygomatic implants have been used to restore function to patients following partial maxillectomies, where all of the support was derived from the zygomatic bone. In light of these developments, the relevance of the alveolar or palatal bone for zygomatic implants is called into question.
Finite element analysis (FEA) has proved a useful tool in the past to investigate the distribution of stresses in and around conventional dental implants. FEA breaks down a complex body into smaller components, each of which can be modelled mathematically. These components are termed elements and are connected by nodes. As forces are applied to the overall body of known material properties, the stresses can be calculated at any given point. This study investigated the importance of the alveolar bone in supporting zygomatic implants using a finite element model.
Materials and methods
Model construction
A computed tomography (CT) scan of a consenting edentulous adult female undergoing zygomatic implant placement was used as the basis for a three-dimensional (3D) model. The CT slices were extracted from the scan using the Mimics software package and thresholded for the Houndsfield values corresponding to bone. In areas where thin bone was present, including the sinuses and floor of orbits, the scan slices were reviewed by the first author, and missed bony outlines were manually drawn in Mimics. A 3D surface model of the left side of the CT scan was then exported as a standard tessellation language file (STL). By only using one side of the skull, models could be created and analysed using fewer elements, thus reducing the model complexity. The STL model was edited using Netfabb, and holes or defects in the surface model were repaired. The repaired STL model was opened in the Rhinoceros software package. Here a series of non-uniform rational B-splines (NURBS) were fitted around the STL surface. The non-uniform rational B-splines surface model was imported to Solidworks as a solid model, which was used for the FEA.
Solid models of the zygomatic implants were created in Solidworks based on the manufacturer’s information leaflets and diagrams. They incorporated a bend at the coronal end to simulate the angled head of the implants. As the implants were going to be continuous with the bone in the FEA process, the threads were omitted. They were placed in the model of the skull so that they penetrated the alveolar bone in the canine and premolar areas and inserted into the zygomatic bone. A bar, 6 mm × 10 mm in cross section, was constructed in Solidworks along the line of the maxillary arch to connect the implants and represent a fixed bridge. The heads of the implants were extended to reach the bridge, representing abutments ( Fig. 1 ).

The skull, implants and bridge were assembled, creating a model of a fixed bridge supported by zygomatic implants ( Figs. 2 and 3 ). This model was duplicated and holes of diameter 5.5 mm were made around both implants as they passed through the maxillary bone. This left a gap of 0.5 mm around the implants in the maxillary alveolar bone, preventing the implants being supported in this area ( Fig. 4 ).



Material properties
The material properties for the skull, implants and bridge were assumed to be homogenous and linearly elastic. The material properties used for bone were derived from averaged values from cadaver studies of the skull. The properties of the zygomatic implants and bridge were based on those for commercially pure titanium as zygomatic implants are made from commercially pure titanium at present. The values used are shown in Table 1 .
Material | Property | Value |
---|---|---|
Bone | Elastic modulus | 1.5 × 10 10 N/m 2 |
Poisson’s ratio | 0.34 | |
Mass density | 1678 kg/m 3 | |
Titanium | Elastic modulus | 1.05 × 10 11 N/m 2 |
Poisson’s ratio | 0.37 | |
Mass density | 4510 kg/m 3 |
Mesh creation and analysis
A mesh was generated from the solid models and consisted of 133,179 elements and 27,455 nodes for the model with alveolar support and 124,256 elements and 26,049 nodes for the model without alveolar support. The superior elements (at the top of the skull) of the model were fully restrained. The medial elements (at the midline of the skull) were restrained using a slider/roller restraint to simulate the presence of the other side of the skull. This allowed movement in the supero-inferior plane and the antero-posterior plane but no medio-lateral movement at the midline. Forces were applied to each model individually in the molar area of the bridge at varying angles to the occlusal plane to assess the effect of changes in force direction. The magnitudes of the forces directed normal to the occlusal plane and at 30° in a buccal and palatal direction were varied to assess the effect of changes in force magnitude. Magnitudes of 50–600 N were analysed. A 3D FEA was run and maximum von Mises stresses were recorded for each model under the various loads. Graphical representations of the von Mises stresses were produced to demonstrate the location of the stresses in the implants, bridge and skull.
Results
The maximum von Mises stresses recorded for each model are shown in Table 2 . The distribution of these stresses in the models and the implants is shown in Figs. 5–10 . In all cases, the maximum stress increased linearly as the applied force was increased ( Fig. 11 ). The maximum stress was located at the head of the distal implant in the model with no alveolar support. In the model with alveolar support, the maximum stress was located at the area where the abutment and bridge met. The stresses applied to the zygomatic bone were low in both models, when compared to the stresses applied to the implants.
Model | Force | 50 N | 150 N | 300 N | 600 N |
---|---|---|---|---|---|
With support | Occlusal | 5.66 | 16.99 | 33.99 | 67.95 |
30° buccal | 5.73 | 17.2 | 34.41 | 68.82 | |
30° palatal | 5.54 | 17.56 | 35.12 | 70.25 | |
No support | Occlusal | 14.2 | 42.59 | 85.18 | 170.36 |
30° buccal | 9.18 | 27.54 | 55.08 | 110.15 | |
30° palatal | 7.29 | 21.86 | 43.72 | 87.44 |


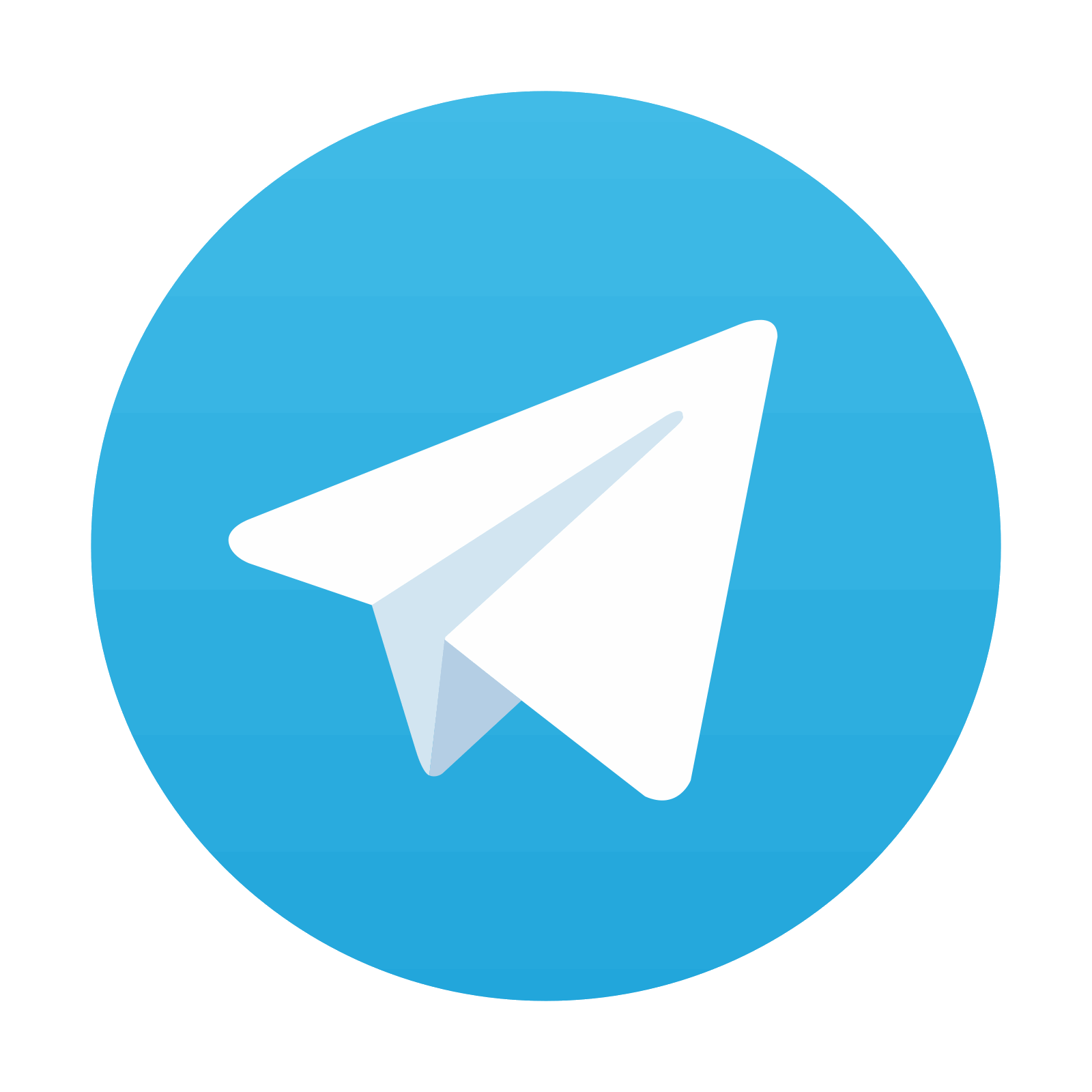
Stay updated, free dental videos. Join our Telegram channel

VIDEdental - Online dental courses
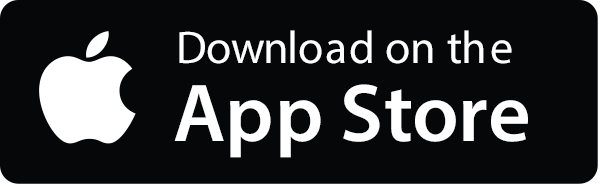
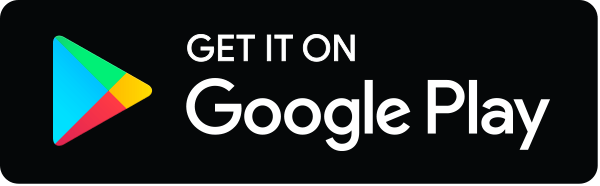
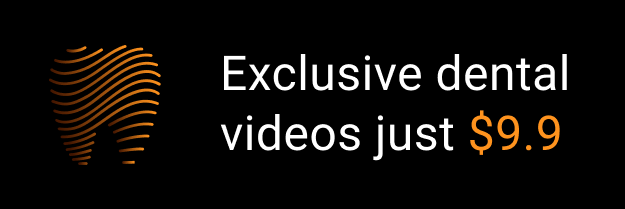