Highlights
- •
A novel method to develop a bone substitute is shown.
- •
Bioactive glasses are used as precursor to form calcium phosphate cements.
- •
Strontium addition in the glass has positive effects on cement’s properties.
Abstract
Objective
The aim of this study was to investigate the effect on properties of increasing strontium substitution for calcium in bioactive glasses used as precursors for novel calcium phosphate cements.
Methods
Glasses were produced by progressively substituting strontium for calcium. Cements were prepared by mixing the glass powder with Ca(H 2 PO 4 ) 2 powder with a 2.5% solution of Na 2 HPO 4 . Setting times and compressive strength were measured after 1 h, 1 day, 7 days and 28 days immersion in Tris buffer solution. X-ray diffraction (XRD), Fourier transform infrared spectroscopy and radiopacity were measured and crystal morphology was assessed using scanning electron microscopy.
Results
A correlation between the phases formed, morphology of the crystallites, setting time and compressive strength were analyzed. Setting time increased proportionally with strontium substitution in the glass up to 25%, whereas for higher substitutions it decreased. Compressive strength showed a maximum value of 12.5 MPa and was strongly influenced by the interlocking of the crystals and their morphology. XRD showed that the presence of strontium influenced the crystal phases formed. Octacalcium phosphate (Ca 8 H 2 (PO 4 ) 6 ·5H 2 O, OCP) was the main phase present after 1 h and 1 day whereas after 28 days OCP was completely transformed to strontium-containing hydroxyapatite (Sr x Ca (10− x ) (PO 4 ) 6 (OH) 2 , SrHA). Radiopacity increased proportionally to strontium substitution in the glass.
Significance
A novel method to develop a bone substitute forming in vitro SrHA as a final product by using a bioactive glass as a precursor was shown. These novel injectable bioactive glass cements are promising materials for dental and orthopedic applications. Further in vivo characterizations are being conducted.
1
Introduction
1.1
Calcium phosphate cements
Calcium phosphate cements (CPCs) are bone grafting materials obtained by mixing one or several reactive calcium phosphate salts with an aqueous solution to form a self hardening paste. These cements are osteoconductive, possess molding capabilities and easy handling properties. They can be injected into bone defects where they set intimately adapting to the bone cavity. They possess sufficient compressive strength when compared to trabecular bone , are non-cytotoxic and have both the chemical composition and an X-ray diffraction pattern similar to those of bone . Poor mechanical properties are the main disadvantages, as the resulting cement is weak under tensile forces and as such they can only be used in combination with metal implants or in non-load bearing applications. They lack macroporosity and as degradation takes place from the external surface, rapid bone ingrowth into the material is impeded. The calcium phosphate salts used as reactive precursors are restricted by their stoichiometry, and therefore alterations of CPCs compositions are limited by the salts used.
1.2
Novel bioactive glass based calcium phosphate cement
Recently a novel injectable bone substitute material has been developed . A bioactive glass (BG) is used as one of the reactive precursor to develop a novel CPC. BGs are amorphous solids produced from a SiO 2 –P 2 O 5 –CaO–Na 2 O system that in contact with physiological fluids form an apatite like layer on their surface allowing bone bonding through interaction with collagen fibrils . BGs are commercially available as bone substitute materials and remineralizing agents in toothpastes . As bone substitutes these materials lack of desirable mechanical properties. They are non-injectable and like other granular bone substitutes they are susceptible to deformation forces until a bond with surrounding tissues is formed. The use of BG as a precursor in CPCs has been shown to improve its properties and overcome the drawbacks of the current CPCs. The BG is a source of Ca 2+ and PO 4 3− ions that react with the Ca(H 2 PO 4 ) 2 salt to form hydroxyapatite (Ca 10 (PO 4 ) 6 (OH) 2 , HA). Using BG introduces the silicate phase which is a potential site for crystal nucleation , leading to a more rapid nucleation than conventional CPCs. Their dissolution in body fluids is correlated to cell mediated and pH dependent mechanisms, thereby undergoing faster resorption rates compared to CPCs. Their dissolution products like soluble silica and calcium ions stimulate osteogenic cells to produce bone matrix . The setting time, strength and resorption rate can be improved by altering the glass composition, with no stoichiometric restriction unlike conventional CPCs. A recent animal study using an ovine model demonstrated that this novel CPC is osteoconductive and successfully osseointegrates with the host bone. It shows a higher degree of osseointegration and new bone formation when compared with a commercial CPC .
1.3
Strontium and its previous uses in CPCs and bioactive glasses
In certain applications, it is desirable to deliver a supply of therapeutic ions in the defect site or to produce substituted apatite phases. Among the bivalent cations that can replace Ca 2+ in the structure of HA, Sr 2+ has attracted a remarkable interest for its biological role. Sr 2+ is present in the mineral phase of the bone, especially at the regions of high metabolic turn-over . Addition of Sr 2+ in biomaterials is beneficial because it has been shown to stimulate cell replication of pre-osteoblasts causing an increase in the rate of new bone formation. Sr 2+ has also been shown to inhibit osteoclast differentiation and activity . Thus, it is reasonable to expect that its presence in bone substitute materials may give better results in terms of biocompatibility and osseointegration. Sr 2+ also adds a degree of radiopacity to the material due to its high atomic number, allowing the implanted material to be observed radiographically . To date, the use of strontium carbonate appears the most suitable choice to obtain a sufficient degree of radiopacity in conventional CPC . In previous works, addition of Sr 2+ in CPCs has been achieved via different methods: (1) producing Sr-substituted salts as the powder starting material ; (2) by adding a separate Sr-containing powder as SrHPO 4 or SrCO 3 ; (3) synthesizing Sr-substituted HA (Ca (10− x ) Sr x (PO 4 ) 6 (OH) 2 ) by a wet chemical route ; (4) adding soluble Sr 2+ salts to the liquid phase used to start the cement reaction . However, this has been shown to have deleterious effect on the setting reactions of CPC . Therefore, a more efficient way is to introduce the Sr 2+ ion in the glass structure enabling the beneficial effect of the ion without adversely affecting the cement properties. Because of their chemical similarity in terms of charge and ionic radius, Sr 2+ for Ca 2+ substitution in BG can be utilized to develop a new glass composition with improved regeneration capability and bioactivity. In vivo bioactive response of a strontium-containing BG is greater due to the biological effect of Sr 2+ on cells and its slightly larger radius than Ca 2+ , which expands the glass network and increases ion dissolution rates . Therefore supersaturation of body fluids with Sr 2+ , Ca 2+ and PO 4 3− will occur faster forming the mixed Ca-Sr HA more quickly. Full substitution of Sr 2+ for Ca 2+ does not result in any decrease in cell proliferation or increased toxicity .
1.4
Aim
In this study a series of Sr 2+ containing BGs were designed and synthesized to produce a range of CPCs by a novel route. The aim of this study was to develop, as the end product of the cement, a n Sr-HA and investigate the effect of Sr 2+ on the physical and chemical properties of the cement.
2
Materials and methods
A previously studied glass with the composition 42.00SiO 2 –4.00P 2 O 5 –39.00CaO–15.00Na 2 O (mol.%) was selected . In all the compositions the network connectivity was kept at 2.00 in order to maintain the same silicate Q structure in the glass network . Sr 2+ was substituted for Ca 2+ with a ratio of 0.05, 0.10, 0.25, 0.50, 0.75 and 1. The glass compositions used are listed in Table 1 .
Glass | % Sr for Ca in the glass | SiO 2 | P 2 O 5 | CaO | SrO | Na 2 O | NC | % Sr for Ca in the cement |
---|---|---|---|---|---|---|---|---|
Sr0 | 0 | 42.00 | 4.00 | 39.00 | 0.00 | 15.00 | 2.00 | 0.00 |
Sr5 | 5 | 42.00 | 4.00 | 37.05 | 1.95 | 15.00 | 2.00 | 2.93 |
Sr10 | 10 | 42.00 | 4.00 | 35.10 | 3.90 | 15.00 | 2.00 | 5.87 |
Sr25 | 25 | 42.00 | 4.00 | 29.25 | 9.75 | 15.00 | 2.00 | 14.70 |
Sr50 | 50 | 42.00 | 4.00 | 19.50 | 19.5 | 15.00 | 2.00 | 29.41 |
Sr75 | 75 | 42.00 | 4.00 | 9.75 | 29.25 | 15.00 | 2.00 | 44.07 |
Sr100 | 100 | 42.00 | 4.00 | 0.00 | 39.00 | 15.00 | 2.00 | 58.81 |
2.1
Glass production and characterization
The glasses were prepared via melt-quench route, by placing a 150 g batch size of mixtures of analytical grade SiO 2 (Prince Minerals Ltd., Stoke-on-Trent, UK), P 2 O 5 , CaCO 3 , Na 2 CO 3 , SrCO 3 (all Sigma–Aldrich, Gillingham, UK) in a platinum-rhodium crucible at 1480 °C for 1 h in an electric furnace (EHF 17/3; Lenton, Hope Valley, UK). After melting, the glasses were rapidly quenched into deionized water to prevent crystallization. After drying, 100 g of each glass was ground using a vibratory mill (Gy-Ro mill/GM100, Glen Creston Ltd., London, UK) for 2× 7 min and sieved using a 38 μm mesh analytical sieve. The amorphous structure of the glasses was confirmed by powder XRD (D8-A25-Advance Bruker; 40 kV/40 mA, Cu Kα). Differential scanning calorimetry (DSC 1500 Stanton Redcroft, Rheometric Scientific, Epsom, UK) was carried out to study the glass transition temperature ( T g ) of the glass powders. Samples were heated from 20 to 1000 °C at 10 °C/min using alumina powder as reference. T g for each glass was then obtained from the corresponding traces, with T g represented by a heat capacity change in slope of the baseline. 5 measurements for each glass were obtained. The particle size distribution of the glass powders suspended in water was determined by laser diffraction analysis by using the principles of light scattering (LS13320 Particle Size Analyser, Beckman Coulter, High Wycombe, UK).
2.2
Ca(H 2 PO 4 ) 2 milling and cement production
The Ca(H 2 PO 4 ) 2 (Sigma–Aldrich, Gillingham, UK) was prepared by grinding 27 g using a vibratory mill (Gy-Ro mill/GM100, Glen Creston Ltd., London, UK) for 4 min. The cement paste was prepared by mixing the sieved glass powder with the milled Ca(H 2 PO 4 ) 2 powder in a ratio to give an overall calcium + strontium to phosphorus ratio (Ca + Sr/P) of 1.3. In order to produce the cement paste and initiate the cement setting reaction the powders were mixed with 2.5% Na 2 HPO 4 solution in a liquid to powder ratio of 0.70 mL/g. The powders were mixed with a spatula on a glass slab and the cement paste left to set in cylindrical steel molds (6 mm height × 4 mm diameter) and placed into a 37 °C oven for 120 min. Seven different compositions of cements were prepared based on the different percentage of Sr 2+ in the glass. Eight samples for each cement composition were made. The calculated percentages of Sr 2+ substitution for Ca 2+ in the cements are listed in Table 1 . The set cement cylinders were then immersed in 10 mL of Tris buffer solution and stored in a 37 °C oven , for either 1 h, 1 day, 7 day or 28 day ( n = 8 for each cement composition and each time point).
2.3
Cement properties characterization
2.3.1
X-ray powder diffraction (XRD)
XRD analysis was performed to check crystalline phases formed (D8-A25-Advance Bruker; 40 kV/40 mA, Cu Kα). The diffraction pattern was taken in the 2 θ range of 3–70 with a step size of 0.001° 2 θ . Crystallite sizes, d , were calculated from the full width at half maximum value (FWHM) at 2 θ around 25.9° corresponding to the (0 0 2) unit cell plane and using the equation:
where λ is the wavelength of the X-rays ( λ = 0.154 nm), k is the broadening constant varying with crystal habit and chosen as 0.9 for the apatite crystallites , w is the FWHM of the peak and θ x the angle of the Bragg reflection.
2.3.2
Compressive strength
Compressive strength was measured using an Instron 5567 mechanical property testing machine (Instron, High Wycombe, UK) with a 1 kN load cell. Force was applied at a movement rate of 1 mm/min. Eight specimens were tested for each cement composition, for each immersion time.
2.3.3
Scanning electron microscopy
The fracture surface of the cement cylinders were studied under SEM. Specimens were gold coated using an automatic sputter coater (SC7620, Quorum Technologies, UK). Photomicrographs were taken using a scanning electron microscope (FEI Inspect F, Oxford Instruments, UK) with the accelerating voltage of 10 kV.
2.3.4
Setting time
Initial and final setting times of the cements were assessed using the Gillmore needle test according to the ISO 9917-1:2007(E) used for dental cement.
2.3.5
Inductively coupled plasma optical emission spectrometry (ICP-OES)
ICP-OES measurements were performed using a Varian Vista-PRO CCD. Samples were diluted by 10 with Tris buffer solution and 69% nitric acid (VWR, Radnor, PA, USA) was added in 2% (w/w). Ion concentrations were measured against a range of prepared multi-element standard solutions (Si, Ca, Na, P, Sr; VWR, Radnor, PA, USA) of 0.1, 1, 10, 20, 50 and 100 ppm, prepared with Tris buffer solution to account for ionic strength. A total of four measurements were performed on each cement immersion time point.
2.3.6
Radiopacity characterization
Radiopacity of cement disk samples (10 mm diameter, 1 mm height) was assessed using a digital dental X-ray system (Heliodent PLUS , Sirona Dental). An aluminum step-wedge used as a reference (Margraf Dental Mfg. Inc.), 9 mm thick with 9–1.0 mm steps, and three specimens for each cement compositions were placed side by side on a periapical imaging plate (Digora imaging plate, size 30 mm × 40 mm, Soredex). The specimens were exposed to X-rays for 0.08 s at 70 kV and 7 mA, with a focus film distance of 10 cm. All plates were processed immediately with a dental imaging system (Digora Optime Classic scanner, Soredex) and visualized with DIGORA (Soredex) software. Radiographic images were treated with Fiji ImageJ software. The average of the gray value of specimens was expressed as equivalent thickness of aluminum in mm.
2
Materials and methods
A previously studied glass with the composition 42.00SiO 2 –4.00P 2 O 5 –39.00CaO–15.00Na 2 O (mol.%) was selected . In all the compositions the network connectivity was kept at 2.00 in order to maintain the same silicate Q structure in the glass network . Sr 2+ was substituted for Ca 2+ with a ratio of 0.05, 0.10, 0.25, 0.50, 0.75 and 1. The glass compositions used are listed in Table 1 .
Glass | % Sr for Ca in the glass | SiO 2 | P 2 O 5 | CaO | SrO | Na 2 O | NC | % Sr for Ca in the cement |
---|---|---|---|---|---|---|---|---|
Sr0 | 0 | 42.00 | 4.00 | 39.00 | 0.00 | 15.00 | 2.00 | 0.00 |
Sr5 | 5 | 42.00 | 4.00 | 37.05 | 1.95 | 15.00 | 2.00 | 2.93 |
Sr10 | 10 | 42.00 | 4.00 | 35.10 | 3.90 | 15.00 | 2.00 | 5.87 |
Sr25 | 25 | 42.00 | 4.00 | 29.25 | 9.75 | 15.00 | 2.00 | 14.70 |
Sr50 | 50 | 42.00 | 4.00 | 19.50 | 19.5 | 15.00 | 2.00 | 29.41 |
Sr75 | 75 | 42.00 | 4.00 | 9.75 | 29.25 | 15.00 | 2.00 | 44.07 |
Sr100 | 100 | 42.00 | 4.00 | 0.00 | 39.00 | 15.00 | 2.00 | 58.81 |
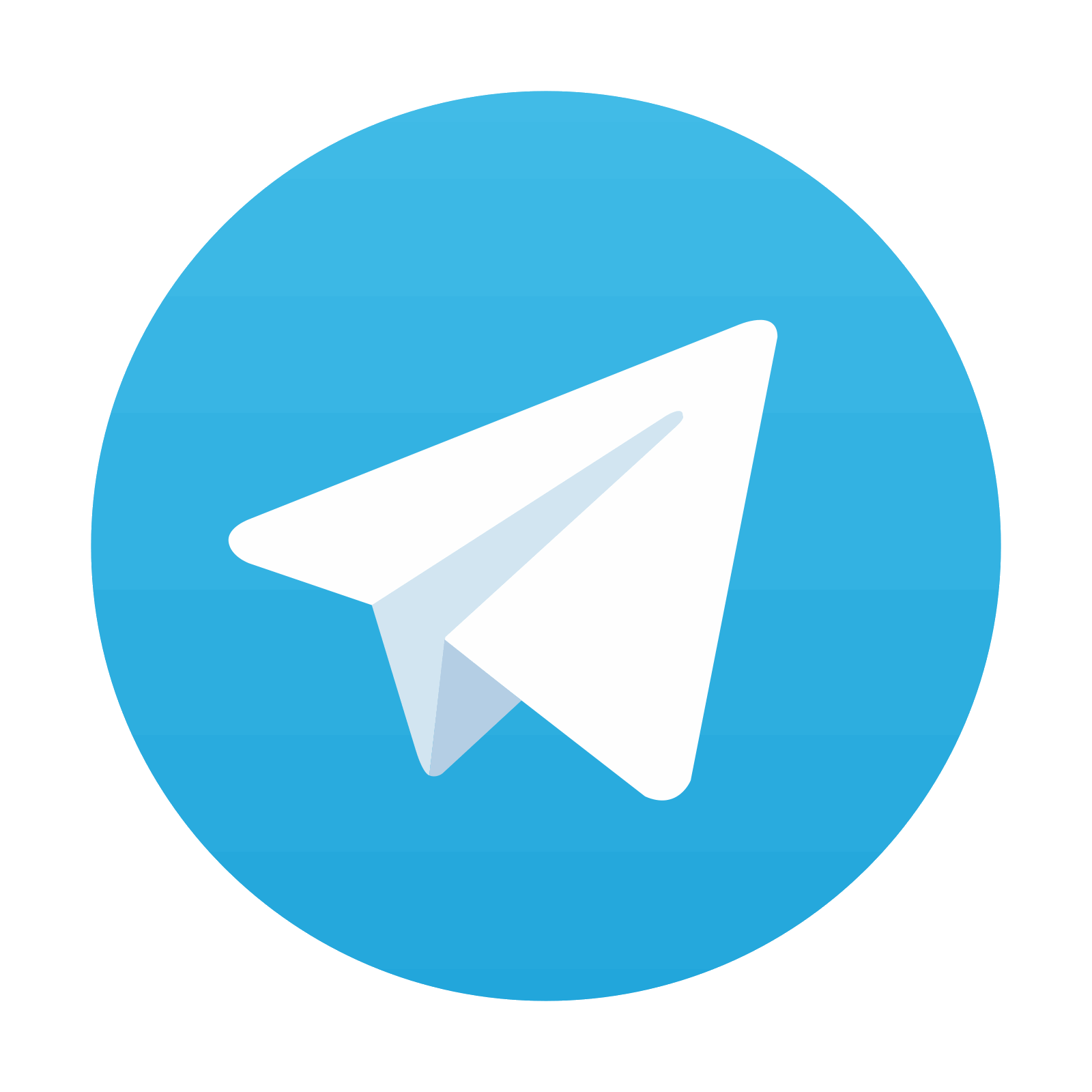
Stay updated, free dental videos. Join our Telegram channel

VIDEdental - Online dental courses
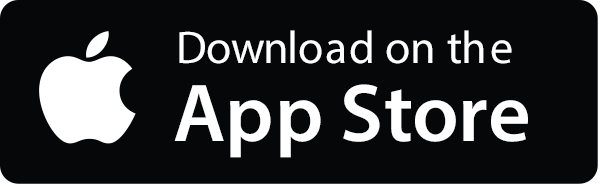
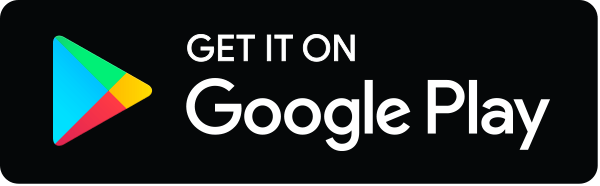
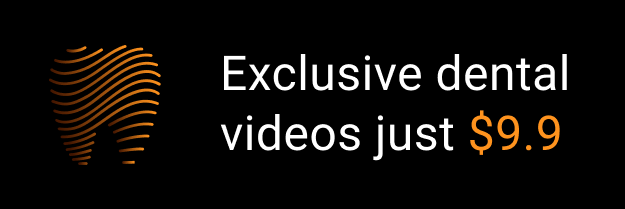