Fig. 4.1
The caries process including demineralization and subsequent remineralization to form a low solubility surface on the crystals [2]
The oral fluids (saliva, biofilm fluid) have calcium (Ca) and phosphate (Pi) in supersaturated concentrations with respect to the mineral composition of enamel. At physiological conditions (a neutral pH of 7), low ion concentrations are sufficient to keep dental hard tissues in equilibrium. If the pH drops because of acid produced by the dental plaque, higher ion concentrations are needed to prevent dissolution of dental hard tissue. Calcium (Ca) and phosphate (Pi) ions are continually deposited on the enamel surface or are redeposited in enamel areas where they were lost. At a pH of ca. 5.5, undersaturation begins, that is, the calcium- and phosphate-ion concentrations in the plaque fluid are not sufficient to maintain the enamel in stable equilibrium; thus, the enamel starts to dissolve.
The term “remineralization” is used to described mineral gain. Remineralization is the body’s natural repair process for subsurface non-cavitated carious lesions [7]. In the process of remineralization, calcium and phosphate ions are supplied from a source external to the tooth to promote ion deposition into crystal voids in demineralized enamel to produce net mineral gain.
De-/remineralization cycles continue in the mouth as long as there are factors including cariogenic bacteria, fermentable carbohydrates, and saliva present. The balance between pathological factors and protective factors determines whether demineralization or remineralization is proceeding at any one time [2].
4.2 Investigations of De-/Remineralization
4.2.1 Models
The de-/remineralization process can be studied using different kinds of models, such as in vitro model, in situ model, animal models, or in randomized controlled clinical trials [8–10]. The induction of artificial carious lesions in bovine and human teeth is an important tool to study strategies for the prevention or treatment of carious lesions. Various models can be selected according to different purposes. Each model has its advantages along with disadvantages. For example, in vitro experiments are the most commonly applied methods. They can be performed over a short period of time, require fewer staff than in situ studies, avoid participant compliance issues, and are relatively inexpensive. But they cannot replicate the oral environment with all of the biological variations known to influence de-/remineralization process [11, 12].
4.2.1.1 In Vitro Chemical Model
The modern pH-cycling models were first produced by ten Cate and Duijsters [13]. In vitro pH-cycling models are widely used, especially for the in vitro evaluation of the efficacy of fluoridated dentifrices for caries control [14]. They are also broadly used in profile studies for rapid and inexpensive testing of developing and recently marketed products [9]. There are several advantages of in vitro pH cycling: (i) the model can mimic the dynamics of mineral loss and gain involved in caries formation; (ii) the high level of scientific control and the resulting lower variability intrinsic to in vitro models; and (iii) it requires smaller sample size [14].
4.2.1.2 In Vitro Biofilm Model
Dental plaque biofilms play a pivotal role in the progression of dental diseases, so in vitro biofilm models are developed to produce artificial caries lesions [15]. Two main aspects should be considered when the most suitable biofilm model for a de-/remineralization investigation was chosen: (1) to select pure cultures or defined communities or microcosms and (2) to select closed system biofilm models or open system biofilm models [15].
Streptococcus mutans are considered the most cariogenic microorganisms in dental biofilm due to their capacity to use dietary carbohydrates to synthesize extracellular polysaccharides (EPS) and because of their acidogenic and aciduric properties [16]. And S. mutans biofilm models as single-species biofilm model are widely used in de-/remineralization investigations [17–19]. Multiple species biofilm models using defined consortia could achieve a high degree of reproducibility between experimental runs which cannot be relied upon when using complex inocula [15, 20, 21]. Microcosms are able to maintain much of the complexity and heterogeneity of the original sample. Saliva was usually collected to form microcosm biofilms to replicate enabling in situ bacterial community dynamics within the laboratory environment [22, 23].
Biofilm models can be divided into two groups according to whether they are “closed” or “open” with respect to nutritional availability [15]. Closed system biofilm models are analogous to batch culture and usually based on multi-well plates [18, 24]. Silva TC et al. applied an active attachment biofilm model as a high-throughput demineralization biofilm model for the evaluation of caries-preventive agents (Fig. 4.2a) [18]. Zürich biofilm model is another typical closed system biofilm models applied in de-/remineralization experiments [21, 25]. Open system biofilm models are analogous to continuous culture. McBain AJ. et al. reviewed different kinds of open system biofilm models [15]. The constant depth film fermentor (CDFF) was widely used for de-/remineralization investigations [23, 26, 27]. The CDFF allows the generation of large numbers of replicate biofilms which can be maintained at a constant depth by static scraper blades (Fig. 4.2b).
4.2.1.3 In Situ Model
In situ models are also widely used for de-/remineralization experiments now. In situ models involve the use of devices creating conditions that simulate the process of dental caries. Enamel and dentin samples are the hard tissue substrates used in in situ models to assess de- and remineralization [8]. The in situ models are designed to simulate the natural process of de-/remineralization and also to provide information in a short period of time without causing damage to the natural teeth of volunteers [28]. These models serve as a link between the clinical uncontrolled situation and the highly controlled laboratory experiments. The in situ caries model designs are highly variable because of the variations of the in situ study designs. All in situ studies must have appropriate controls including a positive and negative control where possible [8].
4.2.1.4 Animal Model
For many reasons—particularly time considerations, animal availability, and attendant costs—rodents have been the most commonly used species for experimental caries studies [21]. According to Stookey et al.’s investigations, the following rat caries models could be used for evaluating fluoride dentifrices: Francis’ hypomineralized area model, Gaffar’s CARA rat model, the Connecticut model, and the Indiana model [29].
4.2.2 Detection and Measurement Methods
Various techniques have been used to investigate the mineral loss and gain during de-/remineralization process, including destructive and nondestructive methods.
4.2.2.1 Transversal Microradiography (TMR)
TMR or contact-microradiography is a highly sensitive method used to measure the morphology of and the change in mineral content of dental hard tissue [30]. But the method is usually destructive to dental hard tissue, so it cannot be used to study any longitudinal mineral changes in exactly the same lesions [31]. To prepare the samples for TMR investigation, thin slices (about 80 μm for enamel samples and 200 μm for dentine samples) are cut perpendicularly to the tooth surface. A microradiographic image is made on high-resolution film by X-ray exposure of the sections together with a calibration aluminum step wedge. The mineral can be automatically calculated from the gray levels of the images of section and step wedge. Parameters of interest are mineral loss (Delta Z in Vol%.μm), lesion depth (Lesd in μm), ratio or average loss of mineral content in the lesion area (Delta Z/Lesd in Vol%), and the mineral vol% and position of the subsurface layer and lesion body [32, 33].
4.2.2.2 Indentation Techniques
Indentation techniques have been used to measure the hardness of the dental hard tissue surface. There are two kinds of indentation techniques for de-/remineralization investigations: microindentation (surface hardness) [32] and nanoindentation (ultra-microindentation) [34]. During the process of both microindentation and nanoindentation, a diamond tip of known dimensions is pressed onto a surface with a given load and duration. The microindentation technique yields data in arbitrary units, usually Knoop hardness number (KHN) or Vickers hardness number (VHN), and nanoindentation yields hardness and reduced elastic modulus in the SI unit of Pascals (Nm−2) [35]. Moron BM et al. revealed that surface hardness analysis should not be interpreted with respect to dentine mineral loss [11]. This was expected as the high organic content, and thus, the elastic properties of the dentine influence the hardness measurement [36].
4.2.2.3 Micro-CT
Micro-CT investigation is a nondestructive method to measure the mineral changes of dental hard tissue. So it is possible to measure and visualize longitudinal mineral changes during de- and remineralization in the same lesion [37]. Published papers proved that micro-CT could offer the quantitative analysis of the de- and remineralization based on CT intensity data [31]. The key point of using micro-CT is to find out how to converse the CT intensity into mineral content. Neves et al. reported a linear correlation between CT intensity (or gray scale value) and the mineral density using three apatite phantoms, the linearity covering a range of 0.25–3.14 g/cm3 [38]. Schwass et al. found a good linearity using six phantoms covering a range from 0.07 to 2.95 g/cm3 [39].
4.2.2.4 Confocal Laser Scanning Microscopy
Confocal laser scanning microscopy (CLSM) is able to identify tissue-emitting fluorescent signal and can be used to detect the mineral loss of dental hard tissue [40–42]. Demineralizing dentin has a strongly increased autofluorescence compared to sound dentine [43]. Some previous studies stained thick enamel samples with a fluorescent dye (0.1 mM rhodamine B) and analyzed using CLSM for quantitating demineralization and remineralization of enamel specimens [44].
4.2.2.5 Quantitative Light-Induced Fluorescence
Quantitative light-induced fluorescence (QLF) is a quantification system for assessing early demineralization or remineralization of human enamel. When high-intensity blue light illuminates the teeth, the resultant autofluorescence of enamel is detected by an intraoral camera. The emitted fluorescence has a direct relationship with the mineral content of the enamel. The intensity of the tooth image at a demineralized area is darker than the sound area. The software of QLF systems can process the image to provide user quantitative parameters such as lesion area, lesion depth, and lesion volume [45–47].
4.2.2.6 Optical Coherence Tomography
Optical coherence tomography (OCT) is a three-dimensional optical imaging technique which works in a similar way to ultrasound, but uses high-frequency light (around 820 nm) instead of high-frequency sound [48]. It is a noninvasive, cross-sectional imaging system that can visualize the internal structures nondestructively [49]. Amaechi et al. developed a quantitative method to detect the demineralizing lesions of dental enamel using an OCT system [50]. This system was able to collect A-scans (depth versus reflectivity curve), B-scans (longitudinal images), and C-scans (transverse images at constant depth). The area (R) under the A-scan could be quantified to indicate the degree of reflectivity of the tissue.
4.3 Methods to Influence the De-/Remineralization Process
4.3.1 Traditional Methods
4.3.1.1 Fluoride
Fluoride was introduced into dentistry over 70 years ago, and it is now recognized as the main factor responsible for the dramatic decline in caries prevalence that has been observed worldwide [51]. Fluoride can be obtained in two forms: systemic and topical. Systemic methods include water fluoridation, salt fluoridation, milk fluoridation, and supplements. Later in the 1940s, the well-conducted water fluoridation program was established in the United States. Though some dentists and researchers have conflicting opinions about their safety and benefits, these systemic methods are still recommended in many countries and receive support from recognized international committees and associations. Due to the widespread application of fluoride and the updated knowledge about its mechanisms of action, topical applications of fluoride (e.g., fluoride toothpastes, gels, varnishes, and mouthwashes) are considered to be more effective methods for caries prevention than systemic use of fluorides [52].
It is believed that fluoride could inhibit demineralization and enhance remineralization [7, 53–55]. Numerous studies were designed to investigate the mechanism of fluoride in inhibiting demineralization of dental hard tissue. According to previous studies, fluoride could incorporate into the enamel apatite structure, enhance the resistance of the dental hard tissue to acidic challenges, and thus inhibit lesion development [7, 56]. In addition, calcium-fluoride-like deposits could form on dental hard tissues and act as a protective barrier on the surface and serve as a reservoir for fluoride [53]. Other researchers have some different opinions. They demonstrate that the incorporation of fluorides into the mineral components of enamel only slightly reduced its solubility [54, 55, 57]. Small amounts of free fluoride ions in solution around the dental hard tissue play a much more important role in inhibiting demineralization. These fluorides have a much greater caries-protective potential than a large proportion of FAP incorporated in enamel mineral [58]. Free fluoride ions are in part adsorbed onto the crystalline surface and are in dynamic equilibrium with the fluoride ions in solution around dental hard tissue. So it forms an equilibrium or supersaturation relative to fluor(hydroxy)apatite, and the adsorption of fluoride on the crystals can offer direct protection from demineralization. Therefore, according to this theory, fluoride should be present in the right place (biofilm fluid or saliva) and at the right time (when biofilm is exposed to sugar or right after biofilm removal), and even small amount of fluoride (below ppm values) available is effective.
In addition to its ability of inhibiting demineralization, fluoride is thought to be effective to promote remineralization. However, fluoride’s ability to enhance net remineralization is limited by the availability of calcium and phosphate ions [59]. If adequate salivary or plaque calcium and phosphate ions are available, fluoride ions can drive the remineralization of extant non-cavitated caries lesions. Fluorhydroxyapatite forms more rapidly even in slightly acidic condition than do the other calcium phosphate phases, so fluoride can accelerate and promote remineralization of dental hard tissue.
It has been demonstrated that fluoride used in enamel remineralization also work in dentine remineralization, and even their remineralization mechanisms are similar [60, 61]. However, dentin demands a considerably higher fluoride concentration in its surrounding solution than enamel does to reach an equivalent degree of demineralization inhibition [62].
4.3.1.2 Calcium Phosphate
Calcium and phosphate ions play important roles in enhancing remineralization of dental caries. Investigators have tried various solutions containing calcium and phosphate ions in their experiments, in which solutions contained between 1 and 3 mM calcium ions with phosphate ions in the ratio of 1:1 [63, 64] or 1.66:1 [63],often with the addition of 1 ppm fluoride ions. Higher concentrations are difficulty to be used because of the instability of the solutions. So the low solubility of calcium phosphates, particularly in the presence of fluoride ions, limited the clinical application of calcium and phosphate remineralization systems. Therefore, novel calcium-phosphate-based delivery systems are developed to combat the demineralization of dental hard tissue. New commercial products are available based on three types of novel systems—crystalline, unstabilized amorphous, or stabilized amorphous formulations.
4.3.2 Novel Methods
4.3.2.1 CPP–ACP and CPP–ACFP
CPP–ACP has been shown to promote remineralization of initial enamel lesions and to prevent demineralization in laboratory, animal, and human experiments [59, 65]. Casein phosphopeptides (CPP) can stabilize calcium and phosphate as nanoclusters of ions through the formation of amorphous nanocomplexes (diameter of 2.12 nm) in metastable solution [66]. But it can also prevent the growth of the nanoclusters to the critical size required for nucleation and phase transformation [65]. CPP contains the active sequence Ser(P)-Ser(P)-Ser(P)-Glu-Glu. Phosphorylated seryl residues are regarded as responsible for the interactions between casein and the calcium and phosphate ions in the nanocomplexes [67].
CPPs can bind to the more thermodynamically favored surface of an apatite crystal face in the caries lesion due to its high binding affinity for apatite. And CPPs prefer binding to the (100) and (010) faces of hydroxyapatite crystals (Fig. 4.3). So crystal growth would be allowed to continue only at the hydroxyapatite (001) plane or along the c-axis, which is the pattern of crystal growth during amelogenesis. Therefore, CPPs are able to regulate anisotropic crystal growth and also inhibit crystal demineralization in the enamel subsurface lesion [68].
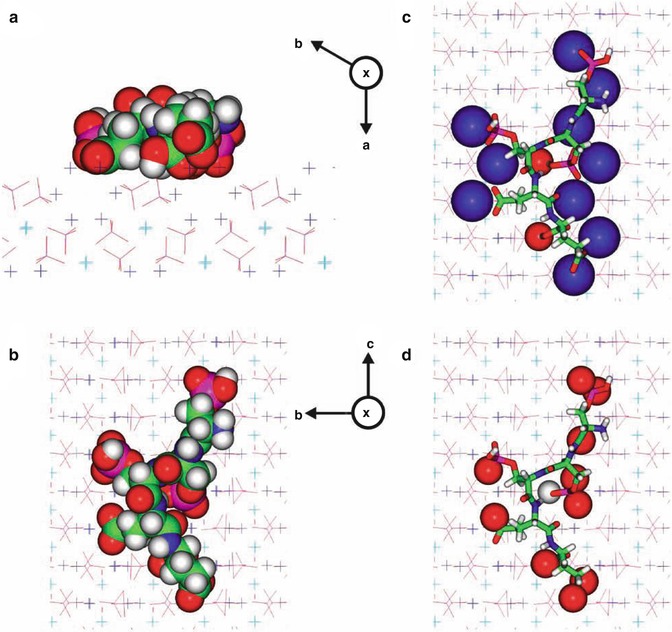
Fig. 4.3
A molecular model of the Ser(P)-Ser(P)-Ser(P)-Glu-Glu motif bound onto the face of hydroxyapatite (HA). The atoms are colorcoded as follows: calcium (1) atoms are light-blue crosses, calcium (2) atoms are dark-blue crosses, oxygen atoms are red, phosphorus atoms are magenta, carbon atoms are green, nitrogen atoms are blue, and hydrogen atoms are grey. The symbol X indicates a crystallographic axis projecting into the paper. Four views are presented: (a) a side view along the c-axis, with the peptide rendered in CPK and the crystal atoms in ‘line’ form; (b) as in (a), but viewed from above, looking down on the HA (100) face; (c) as in (b), with the peptide displayed in stick form and the atoms in the HA surface within 0.25 nm of the peptide rendered in CPK; and (d) as in (b), with the peptide displayed in stick form and the atoms of the peptide within 0.25 nm of the HA surface rendered in CPK. [68]
The amorphous calcium phosphate (ACP) is an important compound because it is a precursor that can convert to apatite, similar to the minerals in tooth enamel and dentin. ACP is an unstabilized calcium and phosphate system. When a calcium salt (e.g., calcium sulfate) and a phosphate salt (e.g., potassium phosphate) are delivered separately, the calcium ions and phosphate ions are mixed and result in the immediate precipitation of ACP or, in the presence of fluoride ions, amorphous calcium fluoride phosphate (ACFP). In the intraoral environment, these phases (ACP and ACFP) are potentially very unstable and may rapidly transform into a more thermodynamically stable, crystalline phase (e.g., hydroxyapatite and fluorhydroxyapatite). However, before phase transformation, calcium and phosphate ions should be transiently bioavailable to promote enamel subsurface lesion remineralization.
4.3.2.2 Natural Medicine
Previous studies indicated some nature medicines were able to influence the de-/remineralization balance of dental hard tissue. The extracts of Galla chinensis (GCE) could inhibit the demineralization and enhance the remineralization of enamel and dentin [69–72]. In addition, this polyphenol compounds had combined effects with traditional remineralizing agents, like fluoride [69], nano-hydroxyapatite [73]. However, the mechanism of GCE is still unclear, and more investigations are still needed.
4.3.2.3 Laser
Several types of lasers, such as erbium-doped yttrium aluminum garnet (Er:YAG) [74, 75], neodymium-doped yttrium aluminum garnet (Nd:YAG) [74, 76], and carbon dioxide (CO2) [76–79], with different parameter settings, have been used for caries inhibition. It is believed that the use of the high-intensity laser on the dental structure can lead to a more acid-resistant surface, and previous investigations showed that lasers could inhibit enamel demineralization and reduce enamel permeability [80].
4.3.2.4 Nanoparticles
Currently, nanotechnology is experiencing rapid growth, with many potential applications in caries prevention and treatment. It is defined as the creation of functional materials, devices, or systems through control of matter on the nanometer scale (1–100 nm). Nanotechnology has motivated mimicking of the nanostructural features of natural human enamel and development of bioinspired strategies for remineralization and caries therapy, respectively [81]. Previous researches have tried to apply nanoparticles in dental caries prevention and treatment, including spherical, cubic, and needlelike nanoscaled particles (approximately 5–100 nm) and near-nanoscaled devices (up to micrometers) [82]. When the sizes of particles are reduced from micrometer to nanometer, the resultant properties can change dramatically. For example, hardness, active surface area, chemical reactivity, and biological activity are all altered [83]. The application of nanoparticles in dentistry can be categorized into two directions: preventive dentistry and restoration dentistry [81].
In recent years, various types of nano-sized hydroxyapatite or calcium carbonate are applied to combat early caries lesions [73, 84, 85]. Some in vitro studies indicated that 10 % suspension of nano-hydroxyapatite particles (10–20 nm diameter, 60–80 nm length) promotes remineralization of the superficial layer of initial caries lesions measuring 20–40 μm, but little remineralization could be obtained by nano-hydroxyapatite in the body of the lesion [73, 84]. Carbonate hydroxyl apatite nanoparticles have also been reported to be effective in repairing micrometer-sized tooth-surface defects in vitro [86]. And some nanocrystals have been incorporated into toothpastes or mouth-rinsing solutions as commercial products.
Clinical investigations indicate that secondary caries and restoration fracture are still the main reasons for dental restoration failure, which limits the longevity of dental restorations, especially the resin composites [87]. Approximately half of all dental restorations fail within 10 years, and replacing them consumes nearly 60 % of the average dentist’s practice time [88]. Investigators try to improve the resin compositions, filler particles, and cure conditions. Calcium phosphate (CaP) particles and calcium fluoride particles have been used as fillers in resin composites (Fig. 4.4). These resin-based CaP or CaF composites can release calcium (Ca), phosphate (PO4), or fluoride ions. These additives enable the resin composite to release calcium and phosphate when the pH is dropped down under in vitro conditions, providing caries-inhibiting properties [90]. These calcium and phosphate or fluoride ion-releasing nanofillers include nanoparticles of dicalcium phosphate anhydrous (112 nm in size), amorphous calcium phosphate (ACP) (116 nm in size), and so on [91–95].
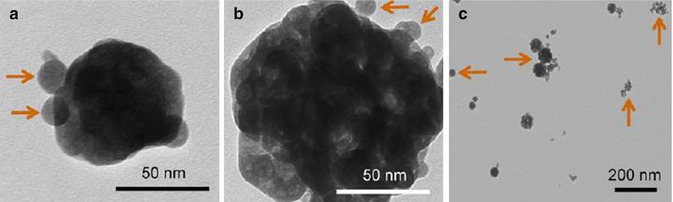
Fig. 4.4
TEM micrographs of the spray-dried nanoparticles: (a) small ACP nanoparticles, (b) ACP cluster, (c) CaF2 nanoparticles [89]
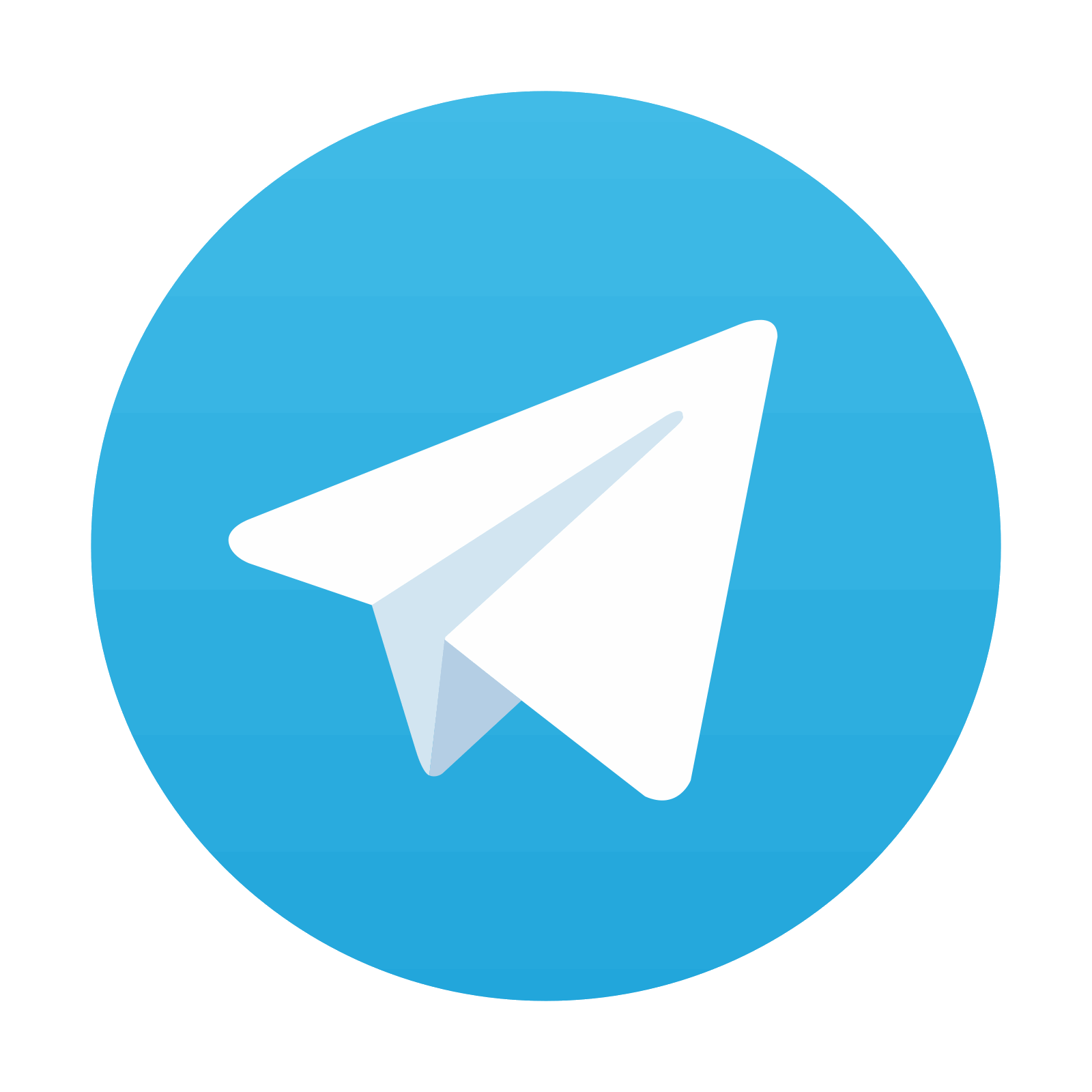
Stay updated, free dental videos. Join our Telegram channel

VIDEdental - Online dental courses
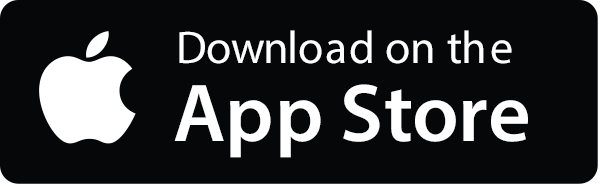
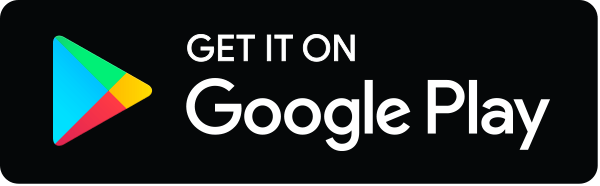