Introduction
Treatment with fixed orthodontic appliances in the corrosive environment of the oral cavity warrants in-vivo investigations of biocompatibility.
Methods
Eighteen control and 28 treated subjects were evaluated longitudinally. Four combinations of brackets and archwires were tested. Buccal mucosa cell samples were collected before treatment, and 3 and 6 months after appliance placement. The cells were processed for cytotoxicity, genotoxicity, and nickel and chromium contents.
Results
In the treatment group, buccal mucosa cell viability values were 8.1% at pretreatment, and 6.4% and 4.5% at 3 and 6 months, respectively. The composite score, a calculated DNA damage value, decreased from 125.6 to 98.8 at 6 months. Nickel cellular content increased from 0.52 to 0.68 and 0.78 ng per milliliter, and chromium increased from 0.31 to 0.41 and 0.78 ng per milliliter at 3 and 6 months, respectively. Compared with the control group, the treated subjects showed significant differences for DNA damage and chromium content at 3 months only.
Conclusions
Fixed orthodontic appliances decreased cellular viability, induced DNA damage, and increased the nickel and chromium contents of the buccal mucosa cells. Compared to the control group, these changes were not evident at 6 months, possibly indicating tolerance for or repair of the cells and the DNA.
The use of various combinations of metal alloys for prolonged durations in orthodontic patients warrants special consideration regarding their biocompatibility. The oral cavity is a complete corrosion cell, with many factors that enhance the biodegradation of orthodontic appliances. Saliva acts as an electrolyte for electron and ion conduction, and the fluctuation of pH and temperature, the enzymatic and microbial activity, and the various chemicals introduced into the oral cavity through food and drink are all corrosion conductors. The inherent heterogeneity of each metal alloy and its use with other alloys, the microsurface discontinuity, the forces acting on the appliances, and the friction between wires and brackets also add to the corrosion process. The literature includes many in-vivo and in-vitro studies documenting the corrosion of orthodontic appliances, and the release of metal ions is indisputable. It has been reported that metal ions are taken up by the adjacent oral tissues.
As pointed out by Wataha, the corrosion of an alloy is of fundamental importance to its biocompatibility because the release of elements from the alloy is nearly always necessary for adverse biologic effects such as toxicity, allergy, mutagenicity, and carcinogenicity. Alloy corrosion provides free ions that affect the tissues around it. There is little evidence that elements released from casting alloys contribute significantly to systemic toxicity. The cause of this might be explained by the low release of ions over time.
Metal tolerance and the amounts causing toxicity are not well understood. Metals are not biodegradable, and their sustained release might produce irreversible toxic effects from their accumulation in the tissues. Also, the increased exposure could limit the recovery time needed for cellular repair. Metal toxicity is governed by multiple factors, making it difficult to truly assess the levels that produce cellular damage.
The general belief that there is no frank concern regarding the corrosion by-products released in orthodontic patients is not actually supported. The literature on cancer research and metal toxicology includes many reports of the dangers of various metal ions. Nickel and chromium ions, which are abundant components of most orthodontic alloys, are classified as chemical carcinogens. The recent insight into the cellular and molecular mechanisms of metal toxicity might cause some concern when dealing with orthodontic appliances. That is due to their direct prolonged contact with the oral tissues and their corrosion, resulting in the release of various types and amounts of metal ions.
Because most research on the amounts of metal ions released from orthodontic alloys has shown that they fall below the recommended daily dietary intakes of nickel and chromium, this might be a false assurance of safety, since chronic low levels of metal ions can alter cellular metabolism and morphology, and produce inflammation and even DNA instability. In addition, some in-vivo studies reported biologic toxicity in orthodontic patients.
To test the biocompatibility (cytotoxic and genotoxic effects on human tissues) of fixed orthodontic appliances, a longitudinal controlled clinical trial was set up with 3 distinct hypotheses: (1) fixed orthodontic appliances do not have a toxic effect, (2) fixed orthodontic appliances do not have an effect on the cellular metal content in buccal mucosa cell samples, and (3) there is no difference between the effects of the various materials of fixed orthodontic appliances.
Material and methods
Sixty subjects were included in this study. Forty patients required fixed orthodontic treatment, and 20 subjects served as the control group. The aims of the study and the method of cell collection were explained to all subjects, and written consent to participate was obtained. Treatment was started after the institutional ethical committee approved the protocol.
Two subjects from the control group and 8 patients dropped out of the study. The causes for not completing the 6-month study period were variable. Some subjects received medications, others underwent oral or general surgeries, others terminated treatment because of discomfort from the appliances, and 4 were excluded for loose brackets. Eighteen control (8 men, 10 women) and 28 treated subjects (6 men, 22 women) completed the study. The average ages were 21 years 6 months (± 3.3 years) in the control group and 20 years 2 months (± 4.4 years) in the treatment group.
The eligibility criteria for subject selection included nonsmokers; no oral diseases, systemic diseases, oral restorations or prosthetics; clinically healthy oral mucosa; no previous orthodontic treatment; no occupational exposure to metals; not receiving any medications or supplements; no radiographic examination during the previous 6 months; and no known allergy to costume jewelry, watches, or sources of nickel and chromium. Subjects were initially screened with a questionnaire to check whether they fit the criteria of the study. They were then clinically assessed for normal oral mucosa. The orthodontic patients were all treated with fixed orthodontic appliances in both arches. The appliances consisted of 4 bands (3M Unitek, Monrovia, Calif) on the first permanent molars, brackets, and 1 type of archwire material throughout the study. The archwires were replaced at 6-week intervals. A total of 4 wires were used for each patient over the 6-month period. The sizes of the archwires were 0.012, 0.014, 0.016, and 0.018 in. The archwires were fixed with 0.01-in stainless steel ligature wire (Leone, Florence, Italy). The compositions of the material alloys are given in Table I .
Material | Fe | Ni | Cr | Mn | Mo | Ti | Co | Cu | Si | Al | P | S | C | O | H | N |
---|---|---|---|---|---|---|---|---|---|---|---|---|---|---|---|---|
Equilibrium titanium bracket (Dentaurum) | ≤0.2 | – | – | – | – | Rest | – | – | – | – | – | ≤0.06 | ≤0.18 | ≤0.13 | ≤0.05 | |
Standard stainless steel bracket (American Orthodontics) | 50–80 | 3–15 | 13–23 | 0–5 | 0–5 | – | 0–5 | 0–5 | 0–5 | 0–5 | – | – | – | – | – | – |
Sentalloy archwire (GAC) | Not available | |||||||||||||||
Stainless steel archwire (GAC) | 69.5 | 9 | 18 | 2 | – | – | 0.75 | – | – | – | – | – | – | – | – | – |
Stainless steel bands (3M Unitek) | Not available | |||||||||||||||
Ligature wire Leowire (Leone) | Rest | 6–8 | 16–18 | ≤2 | – | – | – | – | ≤1 | – | ≤0.045 | ≤0.03 | ≤0.15 | – | – | – |
The patients were divided into 4 groups according to the combination of brackets and archwires. The brackets used were standard stainless steel (American Orthodontics, Sheboygan, Wis) and equilibrium titanium (Dentaurum, Ispringen, Germany), and the archwire materials were stainless steel and nickel-titanium alloys (both, GAC International, Bohemia, NY). Group 1 had stainless steel brackets and stainless steel wires (StSt-StSt). Group 2 had stainless steel brackets and nickel-titanium wires (StSt-NiTi). Group 3 had titanium brackets and stainless steel wires (Ti-StSt). Group 4 had titanium brackets and nickel-titanium wires (Ti-NiTi).
Before the start of the study, all subjects were instructed to continue brushing but not to use toothpastes and mouthwashes containing fluoride or chlorhexidine because these have been reported to increase DNA damage in buccal mucosa cells. A sampling schedule was set up that allowed all subjects to be measured over the same period of time, not consecutively, to prevent the effect of seasonal changes on the assessment of DNA.
Buccal mucosa cells were evaluated before treatment (T0), and at 3 months (T1) and 6 months (T2) after appliance placement. The cells were harvested, according to the method of Nia et al by gentle scraping of the internal part of the right and left cheeks with a wooden tongue depressor. Gentle scraping was required to prevent a heterogeneous cell sample. Each tongue depressor was stirred in a 2-mL tube (Eppendorf, Hamburg, Germany) prefilled with 1.5 mL of ice-cold phosphate-buffered saline solution, free of calcium and magnesium ions at pH 7.4, to detach the cells. Ten strokes on each side were enough to produce adequate cell density in the suspension. The cell suspension was stored on ice in a closed isolated container and immediately transported to the laboratory as recommended by Albertini et al.
Each sample was evaluated for cellular viability by using the trypan blue exclusion dye test. DNA damage was assessed by using the alkaline comet assay according to the protocol of Tice and Vasquez. The lysis step included an additional step of 100 μL of 1 mg per 1 mL of proteinase K for 45 minutes to enhance the lysis step as recommended by Szeto et al. Unwinding was performed for 40 minutes in electrophoresis buffer with the pH above 13 to enhance the expression of minute amounts of single-strand breaks and allow the detection of alkali labile sites to ascertain that negative results were not due to the lack of expression of damage. The electrophoresis unit was set at 20 V for 40 minutes, and the level of the buffer was adjusted until 300 mA was reached. The slides were then electrophoresed in the alkali buffer at room temperature to produce DNA migration. The optimal electrophoretic conditions stretched the supercoiling of the DNA strands and produced migration in approximately 25% of the control cells on a slide. The DNA migration in the control cells provided a guide for the interpretation of comets when lack of comet tails gives false-negative results because of DNA-DNA or DNA-protein crosslinking. The same electrophoretic unit was used throughout the study. The neutralization step was repeated 3 times, leaving the buffer for 5 minutes each time, and the slides were treated with 500 μL of ice-cold absolute ethanol for 10 to 15 minutes. Drying of the gel prevented the diffusion of DNA fragments in the gel during storage. The slides were stored overnight in a light-protected, airtight container at 4°C and scored the next morning by using 50 μL of ethidium bromide (20 μg/mL). Visualization was done in a dimmed room by using an epifluorescent microscope (Leica, Wetzlar, Germany) filled with a green filter (N2.1; excitation filter, BP515-560; dichromatic mirror, 580; suppression filter, LP 590; Leica). A 40-times objective was used. The images were captured with a colored digital camera (DFC280; Leica, Solms, Germany) supplied with Twain imaging software (version 6.11) with host application program imaging (both Leica Microsystem Imaging Solutions, Ltd., Cambridge, UK). Two slides were scored per sample, by grading 50 nucleoids per slide for a total of 100 nucleoids. Only nucleoids of the same size were chosen subjectively for scoring. A grade was given to each nucleoid according to DNA fragmentation in the comet tail. The composite score was then calculated for each sample from the 2 slides. This calculates the DNA damage by multiplying the number of nucleoids in each grade of damage by its grade and summing these values; thus, for each patient at a given sampling time, the composite score was between 0 and 400. Also the damage frequency was calculated; this represents the number of comets per 100 examined nucleoids.
The cellular nickel and chromium contents were measured by using graphite furnace-atomic absorption spectrometry (Varian, Mulgrave, Australia).
The amounts of cell suspension containing 1000 cells were calculated and treated with 100 μL of concentrated nitric acid. The samples were then left for 3 days at room temperature for complete digestion. The sample was diluted online 1 + 4 by the auto sampler with double-distilled water. A normal calibration curve was constructed for each element by 4 points including the calibration blank of the element standard (Merck, Whitehouse Station, NJ) for nickel and chromium to give 0, 2.5, 5, and 10 μg per liter of the standard solutions of the elements. The calibration curve plotted the absorption area signal of solutions of known element concentrations.
Statistical analysis
The statistical analysis was performed with the Statistical Package for the Social Sciences (version 17.01, SPSS, Chicago, Ill). Initially, the control and treatment groups were tested for homogeneity by using the Levene test for the equality of variance. Changes in the treatment and control groups across the time line were evaluated for each variable. The comparisons were done between values at T0 and T1, between T1 and T2, and between T0 and T2. This had the advantage of comparing the same subject with time to limit interindividual variations. Normally distributed variables (composite score and damage frequency) were tested with paired t tests. Viability %, and cellular nickel and chromium contents did not follow a Gaussian distribution and were assessed with the Wilcoxon signed rank test. When the control group showed changes with time, the relative risk was calculated by using the chi-square and Fisher exact tests to compare the incidence of occurrence of a suspected end point in the control and treatment groups. Changes in each test group were also evaluated across the time line with the Wilcoxon signed rank test. Simple linear regression was used to test the effect of cellular metal content (nickel and chromium) on the other variables (viability %, composite score, and damage frequency). The correlation coefficient was calculated for the different variables at the sampling periods (T0, T1, and T2). Measurement of error was calculated for cellular metal content by remeasuring nickel and chromium in 33 randomly selected samples, and the readings were compared with the Wilcoxon signed rank test. All tests were 2-tailed and were evaluated at the 95% CI of the differences.
Results
In the treatment group ( Table II ), the viability % values were 8.1 ± 6.1 at T0 and decreased to 6.4 ± 3.8 at T1. However, a significant decrease was recorded only at T2 (4.5 ± 2.8). The composite score showed a significant decrease at T2 in the treatment group from 125.6 ± 46.05 to 98.8 ± 33.7. The cellular metal content (nickel and chromium) increased significantly. Nickel and chromium at T0 were 0.52 ± 0.32 and 0.31 ± 0.26 ng per milliliter, respectively. The values of nickel at T1 and T2 were 0.68 ± 0.28 and 0.78 ± 0.19 ng per milliliter, respectively, and chromium contents were 0.41 ± 0.28 ng per milliliter at T1 and 0.58 ± 0.26 ng per milliliter at T2.
Test group (n = 28) | |||
---|---|---|---|
Variable | Mean (± SD) | Z | P value |
Viability % | |||
T1 | 6.44 (±3.83) | −1.755 | 0.079 |
T0 | 8.11 (±6.14) | ||
T2 | 4.51 (±2.77) | −2.620 | 0.009 ∗ |
T0 | 8.11 (±6.14) | ||
Cellular nickel content (ng/mL) | |||
T1 | 0.6795 (±0.283) | −4.283 | 0.000 † |
T0 | 0.5196 (±0.316) | ||
T2 | 0.7810 (±0.191) | −3.212 | 0.001 † |
T1 | 0.6795 (±0.283) | ||
T2 | 0.7810 (±0.191) | −3.850 | 0.000 † |
T0 | 0.5196 (±0.316) | ||
Cellular chromium content (ng/mL) | |||
T1 | 0.4090 (±0.276) | −3.645 | 0.000 † |
T0 | 0.3063 (±0.255) | ||
T2 | 0.5839 (±0.257) | −3.121 | 0.002 ∗ |
T1 | 0.4090 (±0.276) | ||
T2 | 0.5839 (±0.257) | −4.146 | 0.000 † |
T0 | 0.3063 (±0.255) |
Mean (± SD) | t | P value | |
---|---|---|---|
Composite score | |||
T1 | 108.4 (±54.06) | −1.351 | 0.188 |
T0 | 125.6 (±46.05) | ||
T2 | 98.8 (±33.70) | −2.814 | 0.009 ∗ |
T0 | 125.6 (±46.05) | ||
Damage frequency (%) | |||
T1 | 45.71 (±17.80) | −0.290 | 0.774 |
T0 | 46.71 (±14.33) | ||
T2 | 42.57 (±10.34) | −1.251 | 0.222 |
T0 | 46.71 (±14.33) |
The control group ( Table III ) showed significant decreases in the composite score and damage frequency at T1. Composite score decreased from 108 ± 30.9 to 50.9 ± 27.1 at T1. The damage frequency decreased from 37.3 ± 11.1 at T0 to 27.4 ± 10.4 at T1. Changes in viability % and cellular metal content (nickel and chromium) were insignificant.
Variable | Control (n = 18) | ||
---|---|---|---|
Mean (±SD) | Z | P value | |
Viability % | |||
T1 | 6.7 (±3.5) | −0.414 | 0.679 |
T0 | 6.2 (±3.5) | ||
T2 | 5.2 (±3.7) | −1.026 | 0.305 |
T0 | 6.2 (±3.5) | ||
Cellular nickel content (ng/mL) | |||
T1 | 0.58 (±0.28) | −1.112 | 0.266 |
T0 | 0.51 (±0.37) | ||
T2 | 0.53 (±0.35) | −1.417 | 0.157 |
T0 | 0.51 (±0.37) | ||
Cellular chromium content (ng/mL) | |||
T1 | 0.29 (±0.16) | −0.283 | 0.777 |
T0 | 0.31 (±0.17) | ||
T2 | 0.29 (±0.12) | −0.632 | 0.527 |
T0 | 0.31 (±0.17) |
Mean (±SD) | t | P value | |
---|---|---|---|
Composite score | |||
T1 | 50.9 (±27.1) | −6.104 | 0.000 † |
T0 | 108 (±30.9) | ||
T2 | 101.1 (±33.6) | −0.836 | 0.415 |
T0 | 108 (±30.9) | ||
Damage frequency (%) | |||
T1 | 27.4 (±10.4) | −3.527 | 0.003 ∗ |
T0 | 37.3 (±11.1) | ||
T2 | 40.2 (±7.6) | 0.777 | 0.448 |
T0 | 37.3 (±11.1) |
The relative risk showed significant differences between the control and treatment groups at T1 for the composite score ( Fig ), damage frequency, and cellular chromium content ( Table IV ). The relative risk at T2 showed no significant differences between the control and the treatment groups for any tested variables.
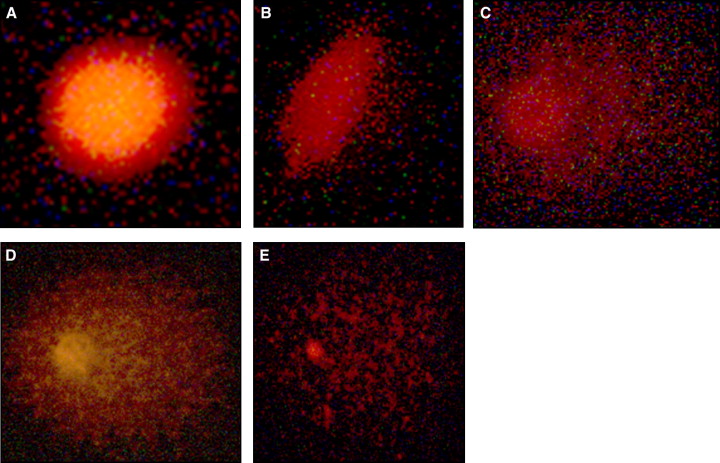
Variable | Treatment period | Relative risk | CI | P value | |
---|---|---|---|---|---|
Composite score | T1 | 0.56 | 0.38 | 0.84 | 0.017 ∗ |
Damage frequency (%) | T1 | 0.54 | 0.30 | 0.97 | 0.04 ∗ |
Cellular chromium content (ng/mL) | T1 | 0.32 | 0.11 | 0.91 | 0.038 ∗ |
Each treatment group was compared with its values at T0 for all tested variables to evaluate any changes.
In group 1 (StSt-StSt), the only toxic changes were decreases in viability % at T1 and T2 ( Table V ). Significant increases in cellular nickel and chromium contents at both T1 and T2 were detected when compared with concentrations at T0 ( Tables VI and VII ). There was no correlation between the increases in nickel or chromium levels and the decrease in viability of the buccal mucosa cells.
Group | Variable | Mean (±SD) | Z | P value |
---|---|---|---|---|
Group 1 StSt-StSt (n = 8) | T1 | 7.8 (±6.4) | −2.111 | 0.035 ∗ |
T0 | 10.2 (±8.0) | |||
T2 | 4.1 (±2.9) | −2.111 | 0.035 ∗ | |
T0 | 10.2 (±8.0) | |||
Group 2 StSt-NiTi (n = 6) | T1 | 5.2 (±2.6) | −0.106 | 0.916 |
T0 | 6.9 (±8.5) | |||
T2 | 4.5 (±2.3) | −0.106 | 0.916 | |
T0 | 6.9 (±8.5) | |||
Group 3 Ti-StSt (n = 6) | T1 | 6.1 (±2.2) | −0.740 | 0.459 |
T0 | 5.5 (±4.2) | |||
T2 | 5.7 (±3.0) | −0.106 | 0.916 | |
T0 | 5.5 (±4.2) | |||
Group 4 Ti-NiTi (n = 8) | T1 | 6.3 (±2.1) | −2.132 | 0.033 ∗ |
T0 | 9.0 (±2.3) | |||
T2 | 4.1 (±3.1) | −2.533 | 0.011 ∗ | |
T0 | 9.0 (±2.3) |
Group | Variable | Mean (±SD) (ng/mL) | Z | P value |
---|---|---|---|---|
Group 1 StSt-StSt (n = 8) | T1 | 0.63 (±0.24) | −2.533 | 0.011 ∗ |
T0 | 0.40 (±0.26) | |||
T2 | 0.76 (±0.27) | −2.111 | 0.035 ∗ | |
T1 | 0.63 (±0.24) | |||
T2 | 0.76 (±0.27) | −2.533 | 0.011 ∗ | |
T0 | 0.40 (±0.26) | |||
Group 2 StSt-NiTi (n = 6) | T1 | 0.57 (±0.17) | −2.220 | 0.026 ∗ |
T0 | 0.43 (±0.18) | |||
T2 | 0.64 (±0.07) | −2.220 | 0.026 ∗ | |
T1 | 0.57 (±0.17) | |||
T2 | 0.64 (±0.07) | −2.220 | 0.026 ∗ | |
T0 | 0.43 (±0.18) | |||
Group 3 Ti-StSt (n = 6) | T1 | 0.91 (±0.44) | −2.220 | 0.026 ∗ |
T0 | 0.69 (±0.51) | |||
T2 | 0.88 (±0.13) | −0.740 | 0.459 | |
T0 | 0.69 (±0.51) | |||
Group 4 Ti-NiTi (n = 8) | T1 | 0.64 (±0.18) | −1.548 | 0.122 |
T0 | 0.58 (±0.23) | |||
T2 | 0.84 (±0.15) | −1.548 | 0.122 | |
T0 | 0.58 (±0.23) |
Group | Variable | Mean (±SD) (ng/mL) | Z | P value |
---|---|---|---|---|
Group 1 StSt-StSt (n = 8) | T1 | 0.44 (±0.13) | −2.533 | 0.011 ∗ |
T0 | 0.29 (±0.08) | |||
T2 | 0.70 (±0.25) | −2.533 | 0.011 ∗ | |
T1 | 0.44 (±0.13) | |||
T2 | 0.70 (±0.25) | −2.533 | 0.011 ∗ | |
T0 | 0.29 (±0.08) | |||
Group 2 StSt-NiTi (n = 6) | T1 | 0.29 (±0.12) | −2.220 | 0.026 ∗ |
T0 | 0.20 (±0.16) | |||
T2 | 0.57 (±0.14) | −2.220 | 0.026 ∗ | |
T1 | 0.29 (±0.12) | |||
T2 | 0.57 (±0.14) | −2.220 | 0.026 ∗ | |
T0 | 0.20 (±0.16) | |||
Group 3 Ti-StSt (n = 6) | T1 | 0.6 (±0.53) | −2.220 | 0.026 ∗ |
T0 | 0.46 (±0.50) | |||
T2 | 0.57 (±0.35) | −1.586 | 0.113 | |
T0 | 0.46 (±0.50) | |||
Group 4 Ti-NiTi (n = 8) | T1 | 0.33 (±0.12) | −0.422 | 0.673 |
T0 | 0.29 (±0.11) | |||
T2 | 0.49 (±0.25) | −1.126 | 0.260 | |
T0 | 0.29 (±0.11) |
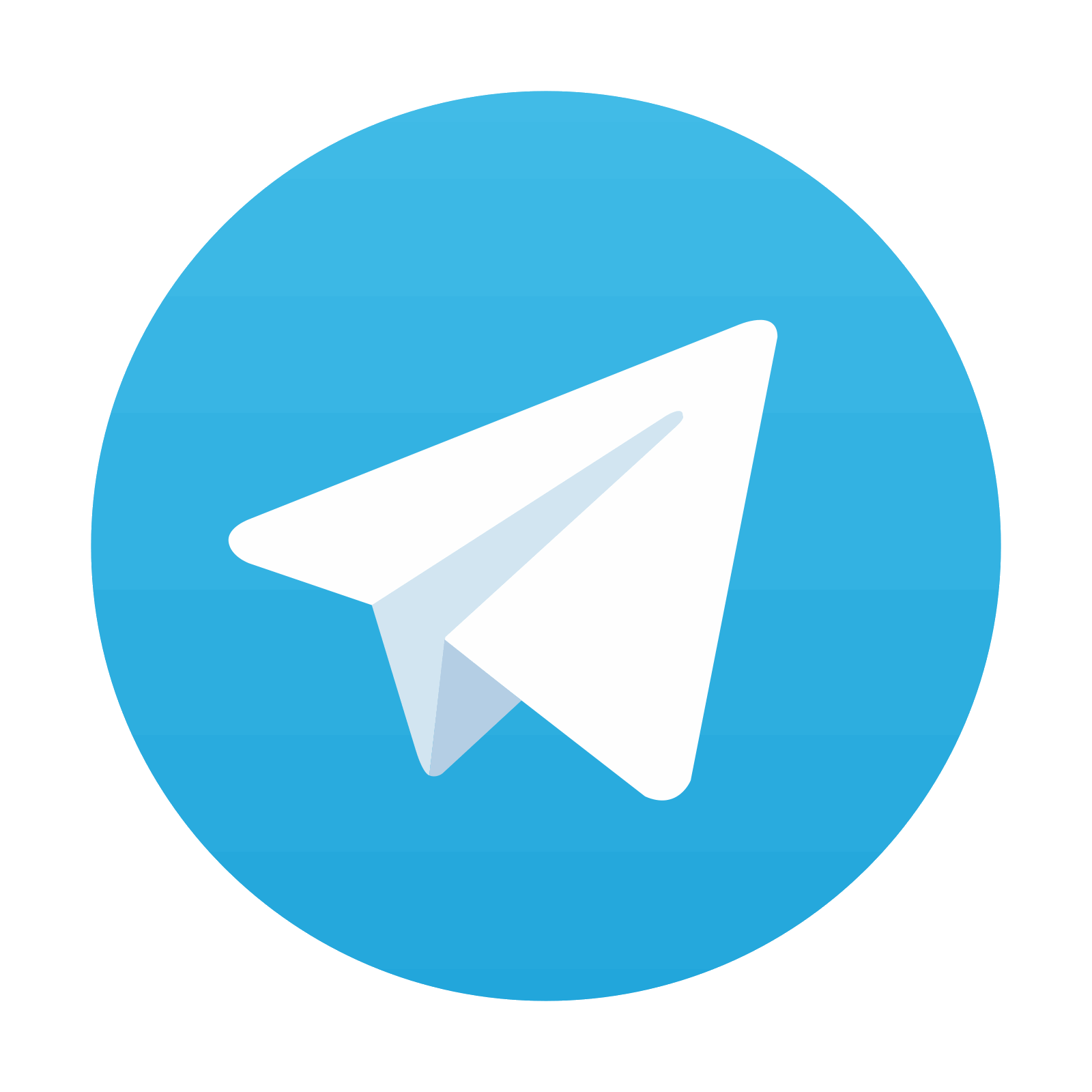
Stay updated, free dental videos. Join our Telegram channel

VIDEdental - Online dental courses
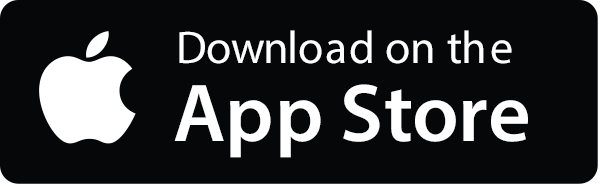
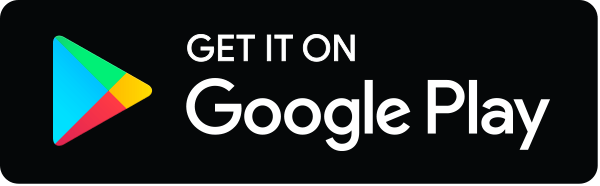
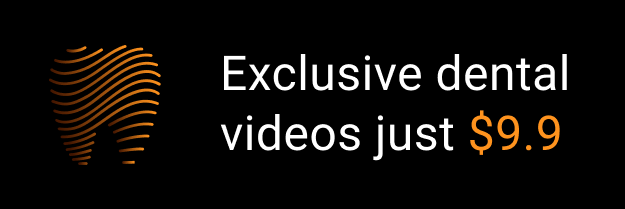