div epub:type=”chapter” role=”doc-chapter”>
K. Orhan (ed.)Ultrasonography in Dentomaxillofacial Diagnosticshttps://doi.org/10.1007/978-3-030-62179-7_3
3. General Considerations for Ultrasound Applications in Head and Neck
UltrasonographyUltrasound probeAdvantages of ultrasoundDisadvantages of ultrasound scanningIndicationsContraindications
3.1 Basics of Ultrasonography

Schematic representation of frequencies of sound related to human and animal hearing range
Contrary to electromagnetic radiation such as X-rays, ultrasound waves require an elastic medium for propagation that can be deformed. When oscillation is transmitted, energy transfer occurs.
The source of ultrasound is a probe, also called a transducer, containing piezoelectric elements usually composed of barium titanate or lead zirconate. These crystals or ceramic elements are characterized by special properties, i.e., when electrical current is applied to the piezoelectric element, it contracts and at the same time emits an acoustic wave. The current is proportional to the force of squeezing. Change of the direction and the focus depth of ultrasound wave is adjusted using the phased array techniques. When the piezoelectric crystal is stretched, the voltage turns to the opposite.

Diagram of fundamentals of ultrasound scanning—echoes return to transducer after bouncing off tissue interfaces at different depths
Acoustic waves are characterized by velocity, wavelength, frequency, and intensity. The velocity equals to the product of multiplication of frequency (in Hertz) and wavelength (in meters). The velocity of ultrasound wave in a human is estimated to be 1540 m/s which is an average value of velocity in tissues, similar to that of an acoustic wave propagated in water. In soft tissues and fluids transmission of ultrasound occurs in the form of longitudinal waves, i.e., the direction of wave propagation is the same or opposite from the direction of the displacement of the medium. In bone, longitudinal wave propagation is accompanied by transverse waves. The velocity of the acoustic wave is characteristic for the medium in which it is propagated therefore when crossing tissue interfaces only wavelength is changed and frequency determined by the source is unaffected [1].
Intensity of ultrasound beam influences the range of examination, i.e., the depth at which imaging still can be performed. Intensity determines the amount of energy transmitted by a wave in one second per unit of area at right angle to the direction of wave propagation.
Ultrasound field generated by a piezoelectric crystal is divided into two parts—near field and far field. In the near field, the width of the beam is constant and the shape of the beam is similar to a cylinder, while in the far field the beam becomes divergent. In the near field, the structure of ultrasound field is not homogenous due to overlapping and interfering of spherical partial waves emitted by different parts of the piezoelectric element. The size of the near field is increased in larger probes, it also increases together with probe frequency.

Physical phenomena encountered in propagation of ultrasound waves in examined object

Example of B-mode ultrasound image demonstrating among others hyperechoic outer cortex of mandibular symphysis (top left corner of the image) with post-acoustic shadowing

Example of B-mode ultrasound image demonstrating among others a hypoechoic, almost anechoic, intraglandular lymph node (marked with calipers) in right parotid gland, with post-acoustic enhancement
A-mode (amplitude mode) is more simple than B-mode as it does not depict distribution of echoes over a two-dimensional cross-section but presents them as plotted amplitude of spikes versus time as a function of depth. In this type of presentation, the probe is placed on skin surface and is not displaced during the examination. Therefore, only mobile objects will produce images in the form of amplitude of echoes. This mode is used in ophthalmology for estimation of distances between different parts of the eye [3].
In M-mode (motion mode, also called Time-Motion mode) the probe is also stationary and only a single chosen ultrasound line is emitted and received. All US reflecting objects are displayed on screen along the time axis. In this type of image, echoes are presented as pixels and their brightness corresponds to the magnitude of echo amplitude. Very high sampling rate in this mode is advantageous as it allows detection and quantification of very fast motions. M-mode is mostly used in cardiology.
Tissue Harmonic Imaging (THI) is based on the properties of nonlinear propagation of ultrasound in the examined tissues. The shape of the ultrasound wave is distorted due to uneven velocity of the wave propagation—i.e., faster high-pressure portion of the wave and slower low-pressure part of the beam. The difference in the form of the wave produces the so-called tissue harmonics which are multiples of the frequency—either fundamental or transmitted. Subsequent harmonics are characterized by decreasing amplitude therefore only the second harmonic is sufficient for generation of an image. THI technique increases signal-to-noise ratio, reduces artifacts coming from reverberations, as well as increases resolution, both axial and lateral.

Diagram of strain elastography—during compression exerted by bouncing of the transducer soft lesions change shape, while firm ones are not remodeled

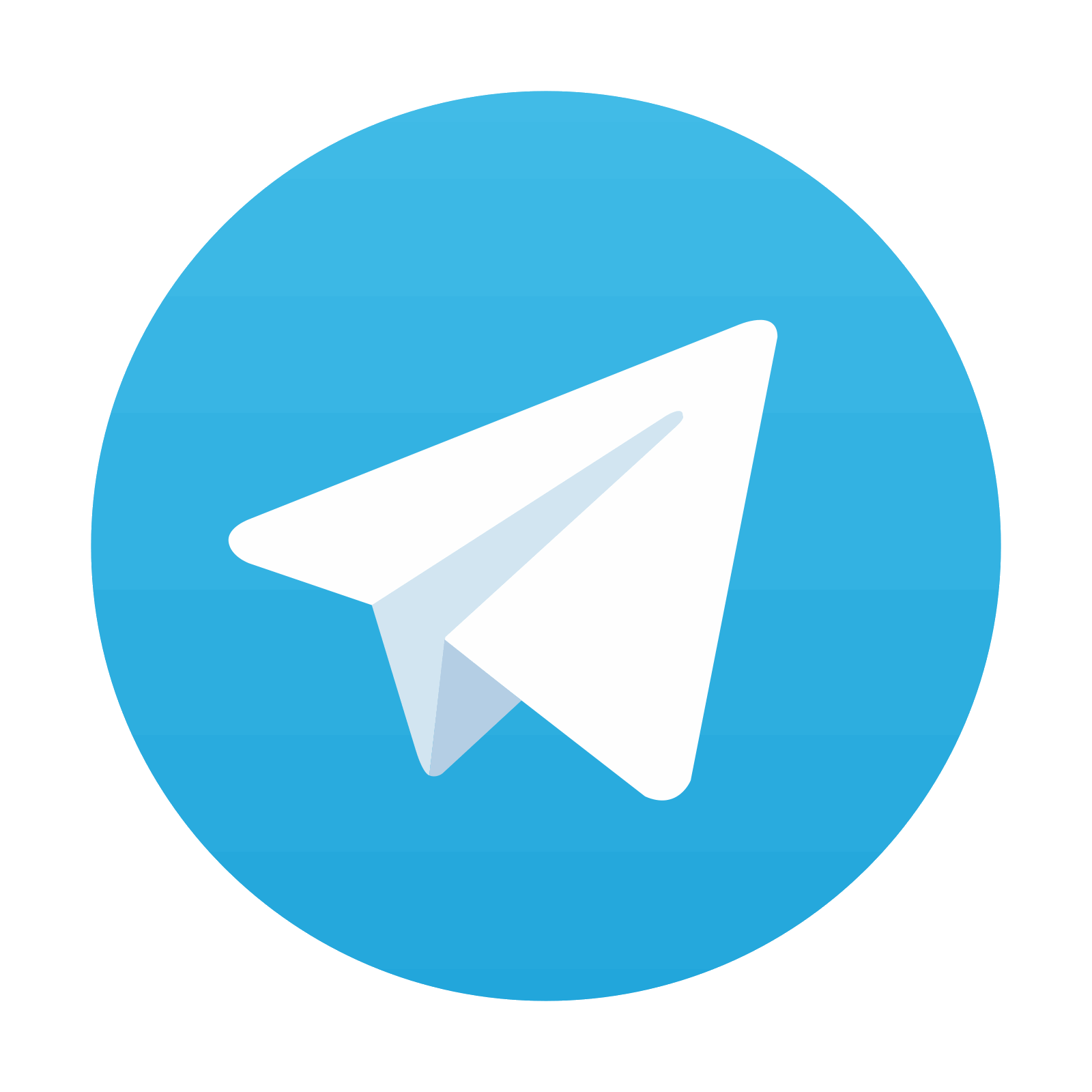
Stay updated, free dental videos. Join our Telegram channel

VIDEdental - Online dental courses
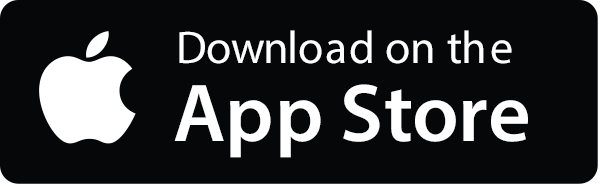
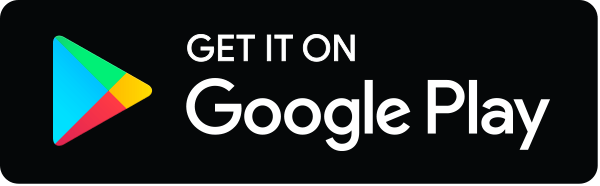