Highlights
- •
The fabrication of novel biomimetic composite materials through a combination of alumina scaffolds and polycarbonate polymer is demonstrated.
- •
Alumina ceramic scaffolds with honeycomb-like microstructures can be fabricated using a gelatine freeze casting technique.
- •
The novel composite materials possess a unique anisotropic and interpenetrating phase microstructure of the honeycomb like alumina ceramic and polycarbonate polymer.
- •
The properties of the novel composite materials produced are found to be advantageous to individual constituents and depend to some extent on the constituent volume percentage.
Abstract
Objective
To determine the mechanical and surface characteristics of two novel biomimetic interpenetrating phase alumina-polycarbonate (Al 2 O 3 -PC) composite materials, comprising aligned honeycomb-like porous ceramic preforms infiltrated with polycarbonate polymer.
Method
Two composite materials were produced and characterised. Each comprised a porous structure with a ceramic-rich (polymer-poor) top layer, graduated through to a more porous ceramic-poor (polymer-rich) bottom layer. In addition, pure polycarbonate and dense alumina specimens were subjected to the same characterisation namely: density, compression, three-point bend, hardness, surface loss and surface roughness testing. Scanning electron microscopy and micro computerised tomography were employed for structural examination.
Results
Three-dimensional aligned honeycomb-like ceramic structures were produced and full interpenetration of the polymer phase was observed using MicroCT. Depending on the ceramic volume in the initial aqueous ceramic suspension, the density of the final interpenetrating composites ranged from 2.64 to 3.01 g/cm 3 , compressive strength ranged from 192.43 to 274.91 MPa, flexural strength from 105.54 to 148.47 MPa, fracture toughness from 2.17 to 3.11 MPa.m ½ , hardness from 0.82 to 1.52 GPa, surface loss from 0.71 to 1.40 μm and surface roughness, following tooth brushing, from 0.70 to 0.99 μm. Composite specimens showed characteristic properties part way between enamel and polycarbonate.
Significance
There was a correlation between the initial solid ceramic loading in the aqueous suspension, used to produce the porous ceramic scaffolds, and the subsequent characteristic properties of the composite materials. These novel composites show potential as aesthetic orthodontic bracket materials, as their properties fit part way between those of ceramic, enamel and polycarbonate.
1
Introduction
There is a growing demand for aesthetic fixed orthodontic appliances, most probably linked to the increasing number of adults who wish to undergo orthodontic treatment, both in the UK and Worldwide . Orthodontic materials currently used to fabricate aesthetic orthodontic brackets include ceramics, e.g. polycrystalline and monocrystalline alumina, and polymers, e.g. polycarbonate and polyurethane. Since their introduction in the 1980s, ceramic brackets have become very popular, with their use in the USA reportedly increasing during the period 1986–2014, from 6% to 70%. At the same time the use of polymeric orthodontic brackets has declined significantly . This is because polymeric brackets suffer from low wear resistance, creep, and discolouration due to water absorption within the oral environment . Although ceramic orthodontic brackets are more popular due to their improved wear resistance and good colour stability , they also have undesirable properties. These include a relatively high hardness, which can lead to excessive wear of the enamel of opposing teeth, their brittle nature, which can lead to unwanted in-service bracket failure, and their high compressive strength, which can result in enamel surface fracture at completion of treatment during bracket removal .
Attempts have previously been made to introduce hybrid ceramic/polymeric brackets, comprising two bulk phases joined at a single interface, in order to create a more durable aesthetic bracket that is easier to debond. The bulk polymer comprises the bonding base and the bulk ceramic the remainder of the bracket. However, the weak link is the single interface between the two bulk materials that often leads to delamination and poor clinical performance . More recently, using the technique of freeze-casting, Alrejaye, Pober and Giordano II fabricated an interpenetrating composite material of alumina and polycarbonate, with toughness and strength values comparable to commercially available alumina ceramic orthodontic bracket materials. This novel composite material shows promise as an aesthetic orthodontic bracket material, not only as a result of the interpenetrating nature of the polymer within the porous ceramic framework, but also because freeze-casting can be used to control the degree of porosity in different parts of the structure during fabrication. The ideal aesthetic orthodontic bracket should be strong enough to withstand the oral environment, be efficient at transferring the applied orthodontic forces to initiate tooth movement during orthodontic treatment, maintain a good appearance/colour and be easy to remove at the completion of treatment with little risk to the enamel surface. The use of a ceramic framework with a graduated porosity interpenetrated by a second polymeric phase has the potential to fulfil these requirements.
The aim of the present study was to characterise novel biomimetic alumina-polycarbonate (Al 2 O 3 -PC) interpenetrating phase composites, produced using the freeze-casting technique followed by heat-pressing infiltration of the polycarbonate (PC) polymer phase. The wear performance of these composite materials was evaluated and compared with their raw constituent materials (alumina and PC polymer), along with human enamel.
2
Materials and methods
Four materials were characterised as part of this investigation. These included, two biomimetic composites produced by freeze-casting aqueous suspensions with either 20% or 30 vol.% initial solid ceramic loadings (Al 2 O 3 -PC-20% and Al 2 O 3 -PC-30%), pure polycarbonate polymer (PC polymer) and densely sintered alumina ceramic. In the case of abrasion testing human enamel was also used for comparison.
In the first step, sintered porous alumina ceramic preforms with different ceramic volume fractions were produced using the freeze-casting method. Initially, ceramic powder was dispersed in water, a dispersant and gelatine and then freeze-cast to create porous green scaffolds. These were then sintered to create ceramic scaffolds with differing porosities, according to the initial solid ceramic loading within the aqueous suspension, as described by Al-Jawoosh et al. .
In the second step, the sintered porous ceramic scaffolds were infiltrated with PC polymer. PC films (Density: 1.20 g/cm 3 , Goodfellow, UK) were hot-heat-pressed into the ceramic preforms at a temperature of 250 °C for 4 h in an oven (Heratherm, Thermo Scientific, UK), using a load of 400N and a stainless-steel cylindrical mould. In each case the weight of the PC polymer was equal to the weight of the porous ceramic preform. Following loading and heat treatment the specimens were left in the oven overnight to cool down before being removed for testing.
Prior to characterisation the Al 2 O 3 -PC composites, pure PC and dense alumina specimens were cut to size using an Accutom-50 (Struers, UK) cutting machine and a diamond saw (Beuhler, USA). The specimen size prepared was dependent on the characterisation test. Once cut, the specimens were polished using a polishing machine (Tegra Pol 15, Struers, UK) with sequential silicon carbide papers under water cooling down to a final 2400 grit. The samples were then placed in an ultrasonic water bath for 30 min (Grant Scientific, UK) to remove any debris and unwanted particles.
For the enamel specimens, third molar teeth were used. Their surfaces were inspected for imperfections and any teeth with cracks, caries, discoloration or loss of hard tissue were excluded. Prior to use the teeth were stored in 0.7% sodium chloride solution containing 0.1% thymol. They were initially sectioned using an Accutom-50 water-cooled high-speed diamond saw (Struers, UK) to separate the crown from the root. Following this step, the pulp chamber was removed from the crown using a high-speed rotatory hand air motor (NSK, Japan). The enamel was then sectioned to produce 2 × 3 × 2 mm sized specimens. Each enamel block was then mounted in epoxy resin (Stycast; Hitek Electronic Materials, Scunthorpe, UK), polished using a polishing machine (Struers, UK) with p1200 silicon carbide discs (Struers, UK), followed by final hand polishing with Al 2 O 3 powder (0.3 μm) as a suspension in deionised water on a glass slab. They were ultrasonicated in deionised water between each polishing stage to remove any debris. The teeth were sourced from an ethically approved tooth tissue bank (REC REF 16/NI/0192) held under HTA licence 12200, project reference: 75.
Once all the composite, alumina and polycarbonate specimens had been prepared, they were characterised as (with the enamel specimens only being used during abrasion and hardness testing) shown in Table 1 .
Material | Density | Compression strength | Flexural strength and modulus of elasticity | Fracture toughness | Hardness | Surface roughness and loss |
---|---|---|---|---|---|---|
Al 2 O 3 -PC-20% | 6 | 6 | 6 | 6 | 6 | 6 |
Al 2 O 3 -PC-30% | 6 | 6 | 6 | 6 | 6 | 6 |
Polycarbonate | 6 | 6 | 6 | None | 6 | 6 |
Dense alumina | 6 | 6 | 6 | None | 6 | 6 |
Enamel | None | None | None | None | 6 | 6 |
2.1
Density
Six rectangular blocks (4 × 4 × 2 mm) of each of the four material types were prepared and their densities obtained using Archimedes’ method, according to ASTM standard, C373–16, USA.
2.2
Compressive strength
Six specimens (4 × 4 × 2 mm) of each of the materials were compression tested using a universal testing machine (Zwick Roell Z020, Ulm, Germany). Once maximum load was reached, the samples were removed and checked to make sure that the fracture point was at the centre of the sample in each case. For this test the composite material samples were divided into two groups. In one group the force was directed parallel to the direction of freeze-casting, with the ceramic-rich layer at the top and the polymer-rich layer at the bottom, and in the second group the force was applied perpendicular to the direction of freeze-casting.
2.3
Flexural strength and elastic modulus
A three-point bend test was used to determine the flexural strength and elastic modulus of each material and in the case of the composite samples this was parallel to the freezing direction with the ceramic rich layer at the top. Each sample (1.8 × 4 × 18 mm) was placed into a computer-controlled universal testing machine according to British Standard, BS EN ISO 6872, 2008 .
2.4
Fracture toughness
Six rectangular specimens of each composite sample (4 × 8 × 32 mm) were polished and pre-notched with a high-speed cutting machine according to the standards for a single-edge-notched beam, ASTM 1820.15.A,USA . A razor blade and diamond paste were then employed to sharpen the notch and extend it an additional 200–350 μm. The final length of the notch was measured with an optical microscope. All the samples were tested using a universal testing machine (Zwick Roell Z020, Ulm, Germany) in the direction parallel to the freeze casting. The notch was made through both the ceramic-rich and polymer-rich surfaces.
2.5
Hardness
Vickers hardness tests were performed at a constant load with a calibrated Vickers indenter in a micro-based indentation system (Duramin Ver 0.08, Struers, UK) according to the standards of Advanced Technical Ceramics, EN843-4: 2005 . Six rectangular specimens (4 × 10 × 12 mm) were subjected to a maximum load for 20 s parallel to the direction of freeze casting on the ceramic-rich surface. Thirty determinations were made for each material using the test method parameters as illustrated in Table 2 .
Parameter | Composite | Enamel | Alumina | Polymer |
---|---|---|---|---|
Hardness | HV 1 | HV 0.2 | HV 2 | HV 0.2 |
Load | 9.807 N | 1.961 N | 19.807 N | 1.961 N |
Indentation diagonals were measured by light microscopy. Any indentations with an irregular shape were rejected. The lengths of the two resultant diagonals of the surface indentations were measured and averaged for each measurement and the hardness calculated.
2.6
Abrasion testing
The aim of abrasion testing was to determine the predicted maximum wear of an orthodontic appliance constructed of a composite material over two years. A custom-made brushing machine (Bristol University) was utilised for brushing the samples using a linear motion (West et al., 2002). Prior to abrasion testing, adhesive tape was used with each specimen to provide two control areas and an approximate 1.5 mm 2 wide surface window exposed to the toothbrushing. A toothpaste suspension was prepared using a ratio of 25 g toothpaste (Colgate Total Everyday, 1450 ppm.F-, 27.6 μmol/L.F-, Colgate-Palmolive Ltd, Guilford, Surrey, UK) to 40 g deionised water, mixed using a stirrer and magnetic flea (Fisher Scientific, Loughborough, UK) until a homogenous suspension was obtained. This was then poured into the brushing machine reservoir to ensure that each specimen was covered by at least 3 mm of dentifrice suspension. The toothbrushes (Oral B, standard medium, size 35, Procter & Gamble, Egham, Surrey, UK) were mounted such that the toothbrush filament tip plane moved back and forth across the specimen. Each test group was allocated a new toothbrush head to avoid contamination between groups. A weight of 200 g was added to the brushes to simulate the everyday brushing force according to International Standard, ISO 11609:2017. The suspension was replaced every hour to ensure adequate coverage of the specimens. The composite specimens were oriented with the ceramic-rich layer at the top, in contact with brush filaments. All samples were brushed for 2 h and 50 min to simulate normal toothbrushing over an average 2-year course of orthodontic treatment. The surface roughness values were evaluated before and after toothbrushing. After brushing, any remaining dentifrice suspension was removed using deionised water before the specimens were then stored in deionised water prior to measurement.
2.7
Surface loss
Non-contact profilometry (Proscan 2100 non-contact profilometer, Scantron Industrial Products Ltd, Taunton, Somerset, UK) and Proscan software (Scantron Industrial Products, Ltd, Taunton, Somerset, England) were used to measure the surface roughness before and after toothbrushing, along with total surface loss after brushing. A dark reference background check was performed each time prior to scanning to achieve optimum sensitivity during measurements.
To determine the amount of surface loss, a 1.5 × 1.5 mm 2 area of each specimen was scanned. The Proscan software was used to highlight three areas in each case, one in the centre across the brushed area (treated) and two from the specimen shoulder areas that were covered and protected initially with tape (controls). This allowed for direct comparison between the brushed and unbrushed areas. Differences in height were calculated using the simulation of the area trace method . The measurements were repeated 3 times and the mean value was considered as the surface loss value of the specimen. Six specimens from each material were investigated. Surface loss was measured by scanning the area that had been brushed (treated) along with the unbrushed areas (control) that had previously been protected by taping.
2.8
Surface roughness
To determine the initial surface roughness before toothbrushing, surface scanning was performed on a scan area of 2 × 2 mm 2 . The optimal step size for the scan area was 0.01 mm in both the horizontal and vertical scan directions and with 200 steps. A reference line was placed on one edge of each specimen in order to record the start position, which was used for all scans with pre-brushing and post-brushing. Six specimens from each material were examined. Surface roughness (Ra, Rz, Rmax and Rq) was measured by scanning the test materials before and after toothbrushing. A S11 chromatic sensor was used for all measurements, with surface filter and auto levelling functions at a sampling rate of 30 Hertz.
2.9
Sample imaging
Samples of the ceramic frameworks and the novel biomimetic composites were imaged using SEM (FEI Quanata 400, FEI, USA) after splutter coating with a gold palladium mixture (Emitech K575X, Quorum Technology Ltd, UK). Samples were also imaged using a MicroCT scanner (Nikon XTH225, Tungsten target, 225 reflection head, Japan) at 120 kV, 300 μA and an exposure rate of 1.4 μm/s, with no filter applied. VGstudio software (VGstudio MAX 3.1, Japan) was used to produce 3D models of the scaffolds from the 2D images obtained by the MicroCT. Rendered 3D images were subsequently obtained using Avizo Standard (version. 8.1, Thermo Fisher Scientific, UK) with isotropic voxels and a spatial resolution of 4 μm.
2.10
Statistical analysis
The data were analysed using Stata version 14 (Stata Corp, College Station, Texas, USA) with a predetermined significance level of alpha = 0.05 and are described in terms of summary statistics.
3
Results
3.1
Microstructure of ceramic preforms and composites
The SEM images in Fig. 1 show the porous ceramic preform made using a 20% initial ceramic loading, before and after infiltration with PC. The pores are characterised by a honeycomb-like structure with good polymer infiltration and no closed pores. Different sizes and shapes of pores were successfully infiltrated with PC.

MicroCT examination was also performed to get a more in depth image of how the polymer phase had infiltrated the porous ceramic scaffolds in all three dimensions. The anisotropic structure can be clearly seen ( Fig. 2 ) with a polymer rich layer at the top and a ceramic rich layer at the bottom with full interpenetration of the polymer into the pores of the ceramic preform.

Fig. 3 shows 3D images of the scanned composite materials. It is clear that the composite comprises a fully interconnected network of a ceramic network phase with almost total interpenetration by the polymer phase. It would seem that increasing the initial solid ceramic loading results in a significant change in the microstructure of the interpenetrating Al 2 O 3 -PC phase composite material. The pores are bigger and more rounded in shape when the initial solid ceramic loading was 20 vol.% compared with the composites produced with 30 vol.% initial solid ceramic loading.

3.2
Density measurements
The mean and SD of the densities of the Al 2 O 3 -PC interpenetrating phase composite materials are illustrated in Table 3 . As might be expected, there is a clear trend of increasing density with increasing initial ceramic content, and between the densities of pure alumina (3.90 g/cm 3 ) and PC (1.20 g/cm 3 ).
Property under test | Al 2 O 3 -PC -infiltrated composite | |||||||||
---|---|---|---|---|---|---|---|---|---|---|
Al 2 O 3 -PC-20% | Al 2 O 3 -PC-30% | |||||||||
Mean | SD | Median | Max | Min | Mean | SD | Median | Max | Min | |
Density (g/cm 3 ) | 2.64 | 0.19 | 2.61 | 3.03 | 2.44 | 3.01 | 0.19 | 2.91 | 3.37 | 2.81 |
Compressive strength parallel to freezing direction (MPa) | 192.43 | 5.97 | 191.93 | 203.73 | 185.10 | 274.91 | 41.05 | 282.67 | 313.41 | 197.61 |
Compressive strength perpendicular to freezing direction (MPa) | 198.26 | 21.81 | 196.70 | 219.90 | 177.77 | 285.15 | 66.91 | 277.77 | 396.95 | 202.70 |
Flexural strength parallel to freezing direction (MPa) | 105.54 | 16.36 | 101.91 | 124.58 | 78.37 | 148.47 | 20.68 | 150.50 | 174.84 | 122.23 |
Modulus of Elasticity (GPa) | 10.72 | 3.85 | 10.50 | 16.58 | 4.14 | 15.17 | 3.22 | 13.58 | 18.65 | 10.73 |
Fracture Toughness (MPa.m½) | 2.20 | 0.16 | 2.23 | 2.41 | 1.99 | 3.11 | 0.32 | 3.01 | 3.79 | 2.76 |
Hardness (GPa) | 0.82 | 0.13 | 0.83 | 0.98 | 0.63 | 1.52 | 0.09 | 1.53 | 1.62 | 1.41 |
Surface loss (μm) | 1.40 | 0.19 | 1.37 | 1.63 | 1.17 | 0.70 | 0.204 | 0.69 | 0.99 | 0.36 |
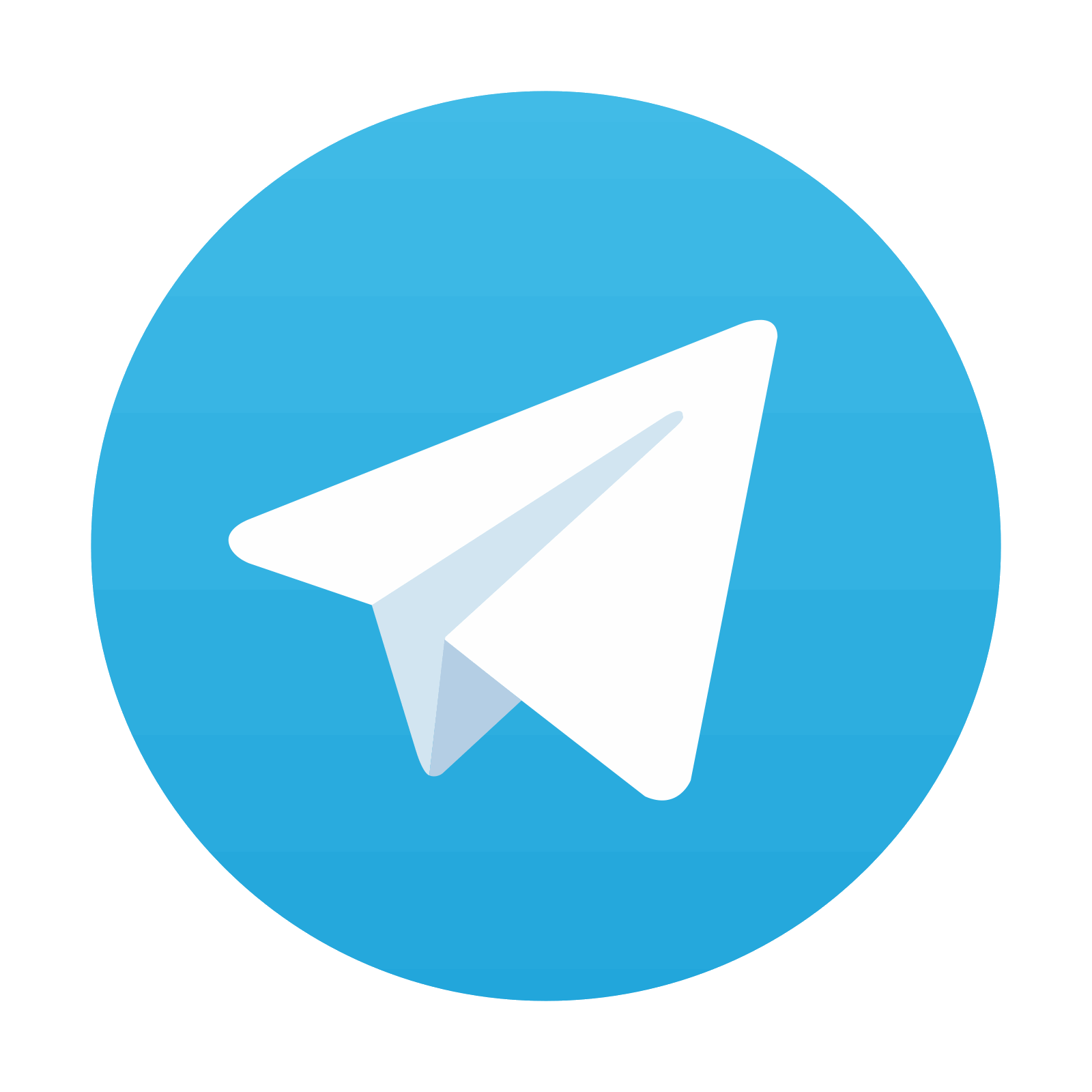
Stay updated, free dental videos. Join our Telegram channel

VIDEdental - Online dental courses
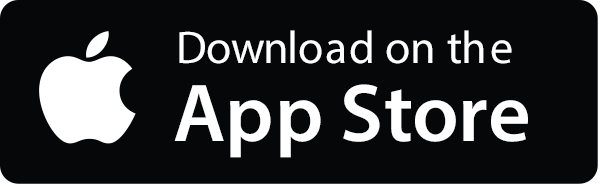
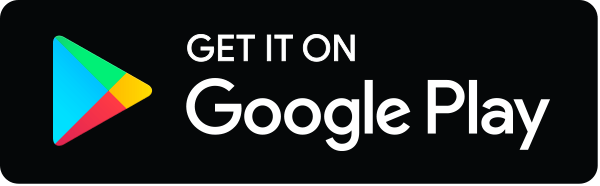
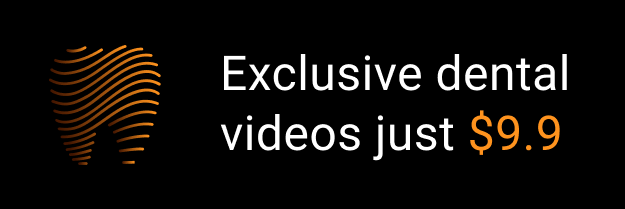