Highlights
- •
Camphorquinone (CQ) induces antioxidant enzymes in a 3D model of the oral mucosa.
- •
CQ is able to pass a dense layer of oral keratinocytes and is metabolized by cells.
- •
CQ alters the transcription and abundance of extracellular proteases.
Abstract
Objective
Objective of our investigation was to determine the influence of CQ on the expression of antioxidant proteins and extracellular proteases in a 3D co-culture model (3DCCM) of the oral mucosa and to analyze the distribution and stability of CQ within 3D-CCMs.
Methods
3D-CCMs consist of confluent keratinocytes (OKF6/TERT2) on cell culture inserts on top of human gingival fibroblasts (HGFs) in collagen. The treatment was carried out by adding CQ to the cell culture inserts at two time points with declining concentrations. Mass spectrometry was used to analyze the CQ concentration above and underneath the OKF6/TERT2-layer. The expression of antioxidant genes was analyzed by qRT-PCR and western blot. The regulation of extracellular proteases from different families was analyzed by qRT-PCR and Proteome Profiler arrays.
Results
GC/MS analysis showed that CQ was evenly distributed within the model. Heme oxygenase-1, NAD(P)H quinone dehydrogenase 1 (NQO1), and superoxide dismutase 1 were induced on the mRNA and protein level in OKF6/TERT2 cells. In HGFs, only the transcription of NQO1 was induced. The transcription of extracellular proteases was increased mainly in OKF6/TERT2 cells 72 h after the initial treatment. The quantity of ten out of 25 analyzed extracellular proteases in the cell culture supernatant above and six underneath the keratinocyte-layer were modulated by CQ.
Significance
Despite its high reactivity, CQ is able to penetrate a dense keratinocyte-layer, presumably across plasma membranes. CQ initially induced the cellular defense machinery against oxidative stress and altered the expression of extracellular proteases. We assume a relationship between both processes.
1
Introduction
Camphorquinone (CQ) is the most commonly used photoinitiator in visible light-curable dental adhesives and composite resins [ ]. CQ generates free radicals when irradiated with blue light between 460 and 480 nm, which initiate the polymerization of monomers like triethylene-glycol dimethacrylate (TEGDMA) or 2-hydroxyethyl methacrylate (HEMA). Photoinitiators and co-initiators are not incorporated into the final polymer network and may leach completely into the oral cavity. Theoretical calculations, based on measured quantities of CQ in various in vitro studies, suggest that CQ concentrations may reach the single-digit mM range locally [ ].
Previous studies have shown that irradiated and non-irradiated CQ causes moderate cytotoxicity in comparison to most other initiators and monomers from dental materials in various cell types in vitro [ , ]. CQ-induced cytotoxicity has been linked to the generation of reactive oxygen species (ROS). Primary human fibroblasts and human gingival fibroblasts (HGFs) exhibited increased intracellular ROS concentrations when treated with irradiated and non-irradiated CQ [ , ]. At the same time, the concentration of the ROS scavenger glutathione was decreased in HGFs when treated with CQ. CQ-treated dental pulp cells (DPCs) showed an increased quantity of heme oxygenase 1 (HO-1) on the mRNA and protein level [ ]. HO-1 produces biliverdin, which is subsequently converted to bilirubin. Both molecules participate in the detoxification of different cellular ROS [ ]. HO-1 is a target gene of the nuclear factor erythroid 2-related factor 2 (Nrf2), a key transcription factor for the regulation of multiple genes that play a major role in the defense against oxidative stress. Other Nrf2-regulated genes with ROS scavenging properties are catalase (CAT), NAD(P)H quinone dehydrogenase 1 (NQO1), and superoxide dismutase 1 (SOD1) [ , ]. NQO1 and SOD1 detoxify superoxide, while CAT detoxifies hydrogen peroxide [ ]. CQ-dependent ROS may also be responsible for the genotoxic and mutagenic potential of CQ as shown by comet assays with CQ-treated HGFs and mouse lymphoma cells [ , ]. Other studies have indicated that the immune status of cells is modulated when treated with CQ. Kim et al. showed that a CQ treatment increased the transcription and extracellular protein amount of the pro-inflammatory cytokines Interleukin-6 and -8 (IL-6, IL-8) in dental pulp stem cells (DPSCs) and also inhibited their odontogenic differentiation [ ]. Chan et al. described that the quantity of one of the key enzymes of inflammatory processes, cyclooxygenase-2 (COX-2), was increased on the protein level when DPCs were treated with CQ. Accordingly, the amount of the inflammatory mediator prostaglandin E2 (PGE2), which is produced by COX-2, was also increased [ ].
Inflammatory processes are frequently linked to a breakdown or reorganization of the extracellular matrix (ECM) [ , ]. Furthermore, extracellular proteases influence inflammatory processes by cleaving extracellular cytokines and chemokines, which regulates their availability and activity [ ]. In addition, extracellular proteases represent a large and diverse group of enzymes that are involved in various molecular mechanisms related to the homeostasis and reorganization of tissues, e.g. cell-cell communication, cell motility, wound healing, embryogenesis, tissue remodeling, etc. [ , ]. Extracellular proteases can be divided into various classes based on their catalytic residue/domain. Prominent classes are metalloproteinases that mostly use zinc as co-factor, and serine and cysteine proteases that use serine and cysteine respectively as nucleophile amino acids to cleave peptide bonds [ ]. Important families of extracellular proteases that contribute to the aforementioned processes are matrix metallopeptidases (MMPs; metalloproteases), cysteine cathepsins (cysteine proteases) and tissue kallikreins (serine proteases) [ ]. To our knowledge, the impact of CQ on the organization of the ECM has not been studied in greater detail. Chang et al. reported that the intracellular quantity of collagen I, an important structural protein of the ECM, was reduced in CQ-treated DPCs [ ]. Kim et al. showed that the amount of MMP-3 was increased in supernatants of CQ-treated DPSCs [ ].
So far, effects of CQ have solely been studied using monolayer cultures with different cell types. However, monolayer cultures may have drawbacks regarding their artificiality in comparison to the in vivo situation. The lack of spatial adhesion domains of stiff plastic surfaces leads to alterations concerning the morphology and physiology of monolayer cultures in comparison to cells grown in 3D matrices [ , ]. Consequently, it has been shown that cells grown in 3D environments behave more similar to tissue in vivo in comparison to monolayer cultures [ , ]. Another drawback of monolayer cultures is the isolated growth of cells. For instance, it has been shown that certain processes, like the balance between MMPs and their correspondent tissue inhibitors of metalloproteinases (TIMPs) or the regulation of differentiation and proliferation is dependent on paracrine interactions between keratinocytes and fibroblasts [ ].
Aim of our study was to expand the knowledge regarding the metabolic effects of CQ on genes related to defense against oxidative stress and extracellular proteases. For that, we used an established 3D co-culture model (3D-CCM) that resembles the oral mucosa, consisting of human gingival fibroblasts (HGFs) grown in a 3D collagen matrix underneath confluent oral keratinocytes (OKF6/TERT2) on cell culture inserts. The porous membrane of the inserts allows for paracrine interactions and enables the separate sampling of both cell types [ ]. Irradiated or non-irradiated CQ was added to the upper compartment (supra-epithelial treatment) at two time points with declining concentrations to mimic the in vivo situation where eluted CQ has to pass the epithelial barrier (keratinocytes) to reach underlying fibroblasts ( Fig. 1 ). This study investigated (1) the effect of irradiated and non-irradiated CQ on the expression of Nrf2-regulated genes, (2) the permeability of a dense cell layer for CQ, and (3) the transcription and protein quantities of extracellular proteases.

2
Material and methods
2.1
Cell cultures
Primary human gingival fibroblasts (HGFs) were obtained from the gingiva of permanent molars from a healthy patient (with informed consent). HGFs were grown in Dulbecco’s modified Eagle’s medium with 4.5 g/L glucose, 25 mM 2-[4-(2-hydroxyethyl)-1-piperazinyl] ethane sulfonic acid (HEPES), 4 mM l -glutamine, 3.7 g/L NaHCO 3 , 100 U/mL penicillin, 100 μg/mL streptomycin, and 2.5 μg/mL amphotericin B, supplemented with 10% fetal bovine serum (FBS, all from Biochrom KG, Berlin, Germany) at 37 °C and 10% CO 2 in a humidified atmosphere. Cells were subcultured by trypsinization with trypsin/ethylenediaminetetraacetic acid (EDTA, 0.25% trypsin, 0.02% EDTA; GIBCO/Invitrogen, Darmstadt, Germany) when the confluency reached approximately 90%.
The immortalized human keratinocyte cell line OKF6/TERT2 was obtained from Dr. J. Rheinwald (Harvard University) [ ]. Keratinocytes were cultured in serum-free medium (ker-sfm) supplemented with 25 μg/mL bovine pituitary extract (BPE), 0.2 ng/mL epidermal growth factor (EGF, all from GIBCO/Invitrogen, Darmstadt, Germany), 0.3 mM CaCl 2 , 100 U/mL penicillin, 100 μg/mL streptomycin, 2.5 μg/mL amphotericin B, at 37 °C and 5% CO 2 in a humidified atmosphere. Trypsin/EDTA (0.125% trypsin, 0.01% EDTA) was used to subculture the cells and trypsin was inactivated by adding one volume of Dulbecco’s modified Eagle medium/Ham’s F-12 medium (DMEM/F-12, Biochrom KG, Berlin, Germany) supplemented with 10% FBS. When a confluency of about 30% was reached, OKF6/TERT2 cells were supplied with medium with a higher nutrient concentration: 1:1 mixture of DMEM/F-12 and ker-sfm supplemented with 25 μg/mL BPE, 0.2 ng/mL EGF, 0.2 mM CaCl 2 , 0.75 mM L-glutamine, 100 U/mL penicillin, 100 μg/mL streptomycin, and 2.5 μg/mL amphotericin B (HD-ker).
2.2
3D co-culture model
3D co-culture models were prepared as described previously with some adjustments [ ]. Briefly, HGF-containing collagen gels (3 mL) were cast on top of an acellular basis (1 mL) in 6-well plates. The cellular gel was prepared from 0.84 mL H 2 O, 0.25 mL FBS, 0.3 mL of 10x DMEM (Biochrom KG, Berlin, Germany), 0.03 mL l -glutamine (200 mM), 0.3 mL 10x reconstitution buffer (20 mM HEPES, 22 mg/mL sodium bicarbonate in 20 mL 0.062 M Sodium hydroxide), 1.18 mL of type 1 rat tail collagen (2 mg/mL in 0.1% acetic acid; SERVA Electrophoresis GmbH, Heidelberg, Germany) and 0.1 mL DMEM with 2 × 10 5 HGFs per gel. After polymerization, collagen gels were covered with 3 mL of DMEM + 10% FBS and cultured at 37 °C and 10% CO 2 in a humidified atmosphere. The HGF-containing collagen gels were continuously observed microscopically and their contraction was measured to ensure consistent seeding and viability of HGFs. 5 × 10 5 OKF6/TERT2 cells in 1.5 mL HD-ker were seeded on a cell culture insert with a pore diameter of 0.4 μm (ThinCert 657641, Greiner-Bio-One, Kremsmuenster, Austria). 2.6 mL HD-ker was added underneath the cell culture insert and the cells were cultivated at 37 °C and 5% CO 2 in a humidified atmosphere. HGFcontaining collagen gels and OKF6/TERT2 cells were cultivated separately, until 3D-CCMs were assembled seven days after seeding. For that, both components were transferred to a new 6-well plate and supplied with 2.6 mL of DMEM/F-12 medium supplemented with 25 μg/mL BPE, 0.2 ng/mL EGF, 0.2 mM CaCl 2 , 1.5 mM l -glutamine, 100 U/mL penicillin, 100 μg/mL streptomycin, 2.5 μg/mL amphotericin B and 5% FBS (DF-K medium) in the lower compartment and 1.5 mL of DFK with the test substance as described in chapter 2.4 in the upper compartment. Assembled 3D-CCMs were cultivated at 37 °C and 5% CO 2 in a humidified atmosphere. Lucifer Yellow assays were carried out using extra cell culture inserts with OKF6/TERT2 cells before the assembly to ensure that keratinocytes reached the desired confluency in every experiment [ ]. Briefly, the strongly hydrophilic fluorescent dye Lucifer Yellow was added to the upper compartment, above OKF6/TERT2 cells. The amount of Lucifer Yellow that was able to pass the cell layer was measured in the lower compartment and calculated relative to the theoretical equilibrium concentration. A threshold of 8% Lucifer Yellow of the equilibrium concentration was defined previously as a threshold for a maximal confluency of OKF6/TERT2 cells on cell culture inserts, which was optically supported by microscoscopy [ ].
2.3
CQ Treatment of 3D co-culture models
The photoinitiator CQ was supplied by VOCO (Cuxhaven, Germany) and solved in ethanol. 3D-CCMs were treated only in the upper compartment (supra-epithelial treatment) at two time points. 2.5 mM CQ in 1.5 mL DF-K medium was added to the upper compartment and 2.6 mL DFK medium to the lower compartment when the 3D-CCMs were assembled. After 24 h, half of the 3D-CCMs were harvested and the treatment solution of remaining models was exchanged with fresh treatment solution in the upper compartment and DFK medium in the lower compartment. The second treatment was carried out with 50% of the initial CQ concentration (1.25 mM). After additional 48 h, the remaining 3D-CCMs were harvested (72 h time point). The concentration of the solvent (ethanol) was equal in every treated sample at all phases of the experiment. In one variant, the treatment solution was irradiated with blue light two times for 10 s directly after the addition to the upper compartment using a dental curing light (800 mW/cm 2 ; Elipar II, Espe, Seefeld, Germany). 0.25% ethanol (C1), 0.25% ethanol with blue light irradiation (C1 + BL) and culture medium (C2) served as controls. A schematic presentation of the model and the experimental setup is shown in Fig. 1 .
2.4
Gene expression analyzes
RNA isolation, cDNA synthesis, and gene expression analysis of genes related to oxidative stress and extracellular proteases was carried out as described previously [ , ]. The QuantiTect Primer assays used are listed in Supplemental file 1 (Qiagen, Hilden, Germany). We applied geNorm to evaluate the stability of six candidate housekeeping genes for both cell types independently (18S ribosomal RNA [ 18S rRNA ], Actin beta [ ACTB ], Beta-2-microglobulin [ B2M ], Glyceraldehyde-3-phosphate dehydrogenase [ GAPDH ], Succinate dehydrogenase complex subunit A flavoprotein [ SDHA ] and Tyrosine 3-monooxygenase/tryptophan 5-monooxygenase activation protein zeta [ YWHAZ ]) [ ]. B2M and YWHAZ were identified as most stable housekeeping genes for OKF6/TERT2 cells and GAPDH and YWHAZ for HGFs. Normalized ratios between samples and the untreated control (C2) were calculated using the efficiency adjusted delta delta ct method according to Pfaffl [ ]. Three independent sets of experiments were performed.
2.5
Western blot
Isolation of total cellular proteins and western blots were carried out as described previously [ ]. The following primary antibodies were used: anti-GAPDH (rabbit; 1:10,000 in PBST with 0.5% milk powder; Sigma-Aldrich, St. Louis, USA), anti-HO-1 (rabbit; 1:2,000; Enzo Life Sciences; Farmingdale, USA), anti NQO1 (goat; 1:1,000; Abcam, Cambridge, UK) and anti-CAT (rabbit; 1:16,000; Merck, Darmstadt, Germany). Goat anti-rabbit or rabbit anti-goat, conjugated with horseradish peroxidase were used as secondary antibodies (1:10,000 in PBST with 0.5% milk powder, DakoCytomation, Hamburg, Germany). Polyvinylidene difluoride membranes (PVDF, Millipore, Burlington, USA) were developed with an enhanced chemiluminescence substrate (SuperSignal West Pico chemi-luminescent substrate, ThermoFisher, Waltham, USA). The chemiluminescence signal was captured using an imaging system (Fusion SL-4-400WL, Vilber, Eberhardzell, Germany) and quantified with the software Bio1D (Vilber Lourmat). Experiments were carried out three times independently.
2.6
GC/MS measurement of CQ
Cell culture inserts with OKF6/TERT2 cells were treated with 2.5 mM CQ or 0.25% ethanol (C1) as described in 2.3. 1 mL medium was taken from the upper and the lower compartment at both time points (24 h and 72 h) and briefly centrifuged. The supernatant was used for GC/MS measurements. For a more detailed analysis of the kinetic of the CQ allocation within the first 24 h, six inserts with OKF6/TERT2 cells were treated with 2.5 mM CQ. 1 mL medium was taken from each compartment at standardized time points (0.5 h, 1 h, 3 h, 6 h 12 h 24 h) and prepared as described above. Two inserts with OKF6/TERT2 cells were treated with 0.25% ethanol and sampled at the time points 0.5 h and 24 h as solvent controls (C1). Additionally, an aliquot of the 2.5 mM CQ treatment solution was incubated in 6-well plates without cells at 37 °C and 5% CO 2 in a humidified atmosphere to evaluate the influence of time and temperature on the CQ degradation in medium. Eight calibrators were prepared by consecutively diluting the 2.5 mM CQ treatment solution 1:2 with DFK medium supplied with 0.25% ethanol to keep the ethanol concentration constant. CQ was extracted by adding one volume of ethyl acetate (Merck, Darmstadt, Germany), supplemented with 1.25 mM caffeine (SigmaAldrich, St. Louis, USA) as internal standard, followed by 10 min of mixing. Additional 0.5 vol of ethyl acetate was added afterwards. After 2 min of vortexing, the mixture was centrifuged at 5445× g (5 min, 4 °C). 50 μL of the resulting upper phase were transferred to an amber glass vial with inserts (f N11-1 HP; MachereyNagel, Düren, Germany) and 200 μL ethyl acetate was added. 1 μL of each extract was injected via a 7693 ALS Autosampler (Agilent Technologies, Santa Clara, USA) into the Agilent 7890B GC System equipped with a HP-5MS UI column, 30 m × 0.25 mm ID, 0.25 μm film thickness coupled to an Agilent 7000C GC/MS triple quadrupole mass spectrometer. Helium gas with a constant flow of 1.2 mL/min was used as carrier gas. The following temperature program was used for the separation in the MS: 50 °C initial temperature for 1 min followed by a temperature-ramp of 25 °C/min to 150 °C and a second ramp of 15 °C/min to 300 °C, held for 1 min. The resulting retention time of CQ was 5.84 min. CQ was identified by MS via its m / z 69.1 (quantifier ion) and m / z 86.9 (qualifier ion) mass fragments. Three independent sets of experiments were performed.
2.7
Proteome Profiler array
The Proteome Profiler array ‘Human Protease Array Kit’ from R&D Systems (Minneapolis, USA) was used to quantify extracellular proteases in supernatants of 3D-CCMs that were used in experiments for western blot analysis. Samples were taken from both compartments of 3D-CCMs that were treated with 0.25% ethanol (solvent control; C1) or with CQ without blue light irradiation at the second harvest time point, 72 h after beginning of the treatment (described in Section 2.3 ). The cell culture supernatant was taken before the cells were harvested for protein analysis and briefly centrifuged to pellet floating cells and cell debris (5 min; 800× g ). The resulting supernatant was transferred to a new tube and stored at −80 °C. The Proteome Profiler array was performed according to the manual, using 400 μL of each supernatant. The chemiluminescence was captured using a Fusion SL-4-400WL imaging system. The spot intensities were quantified based on pixel densities with the software Bio1D. The signals were normalized by background subtraction and quantified in relation to the positive controls of the membranes. Spots that showed signal intensities only slightly above the background and also spots that were influenced by very bright spots next to them were discarded from the analysis. Experiments were carried out three times independently. Representative membranes are shown in Supplemental file 2.
2.8
Statistics
Fold changes from qRT-PCR and western blot analyzes ( Fig. 2 and 4 ) were calculated relative to the medium control (C2) and log2transformed to enable statistical testing. The values from treated samples were tested against their corresponding solvent control (C1) from the according time point (24 h/72 h: CQ vs C1 and CQ + BL vs C1 + BL) via two-tailed t-test with a significance level of 0.05. The same test was applied when testing the results from GC/MS analyzes comparing CQ concentrations between the upper and lower compartment ( Fig. 3 ). For Proteome Profiler arrays ( Fig. 5 ) and the comparison of results from Proteome Profiler arrays to the gene expression analysis of MMPs ( Fig. 6 ), ratios from normalized signal intensities were calculated relative to the solvent control (C1). Ratios were log2-transformed and tested by one sample t -test to be significantly different from 0 (not regulated) in consideration of the variance from 3 biological replicates. The correlation between the regulation of the transcription of genes from both cell types and the protein quantities of extracellular proteases in the cell culture supernatants from both compartments ( Fig. 6 ) were calculated using the Pearson correlation coefficient (based on the linear values).

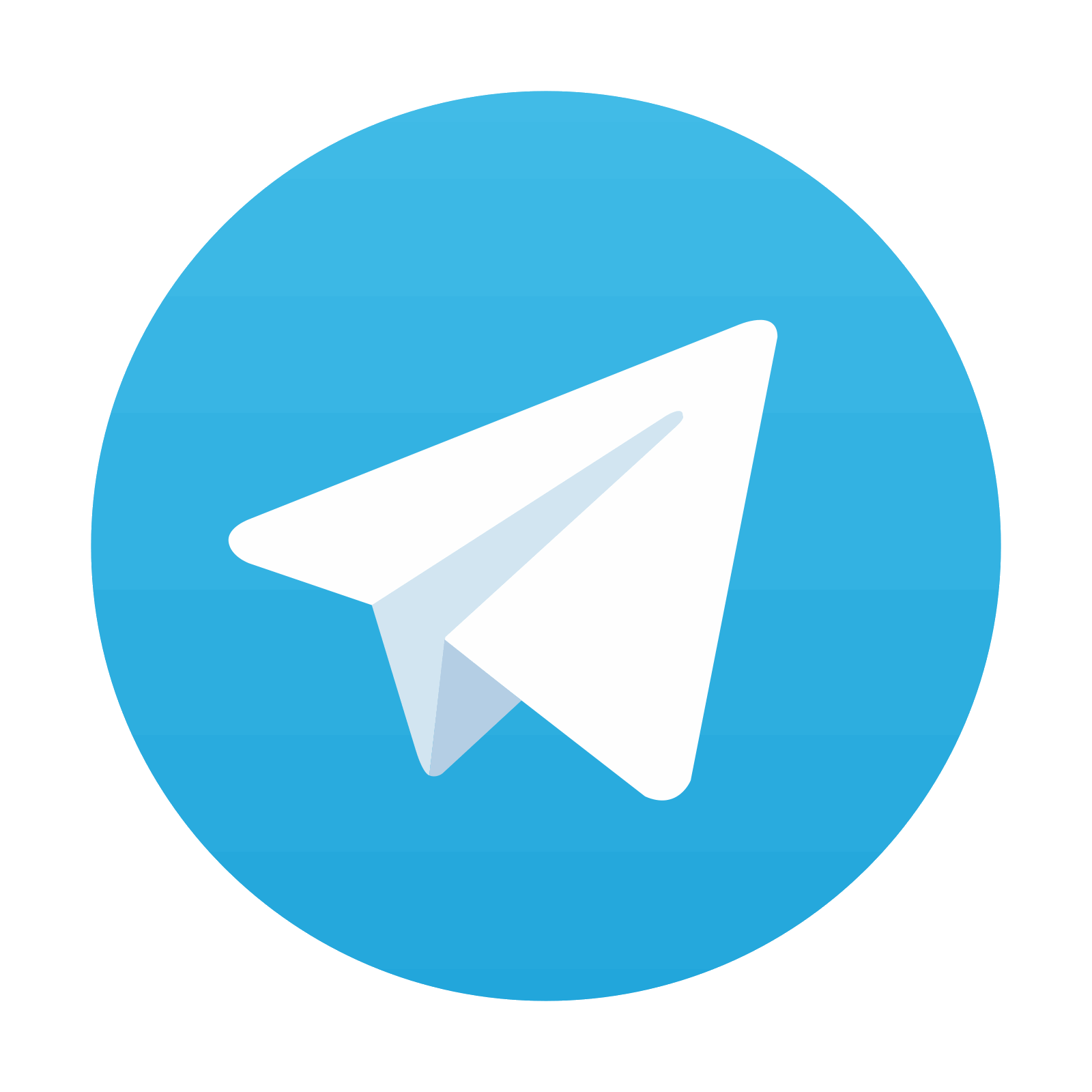
Stay updated, free dental videos. Join our Telegram channel

VIDEdental - Online dental courses
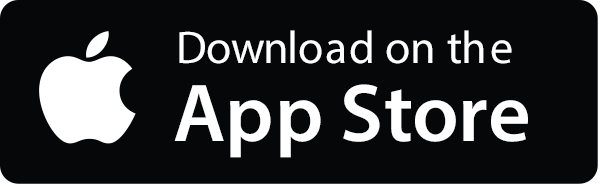
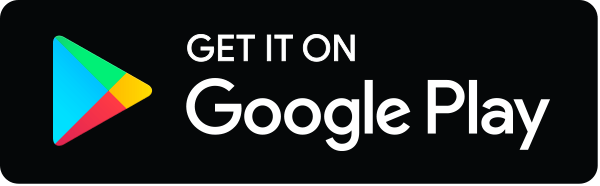
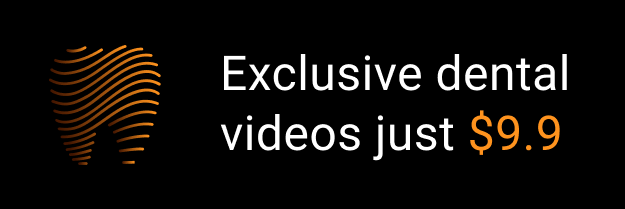