Abstract
Objectives
The aim of this study was to assess the effect of abutment’s diameter shifting on reliability and stress distribution within the implant-abutment connection for internal and external hexagon implants. The postulated hypothesis was that platform-switched implants would result in increased stress concentration within the implant-abutment connection, leading to the systems’ lower reliability.
Methods
Eighty-four implants were divided in four groups ( n = 21): REG-EH and SWT-EH (regular and switched-platform implants with external connection, respectively); REG-IH and SWT-IH (regular and switched-platform implants with internal connection, respectively). The corresponding abutments were screwed to the implants and standardized maxillary central incisor metal crowns were cemented and subjected to step-stress accelerated life testing. Use-level probability Weibull curves and reliability were calculated. Four finite element models reproducing the characteristics of specimens used in laboratory testing were created. The models were full constrained on the bottom and lateral surface of the cylinder of acrylic resin and one 30° off-axis load (300 N) was applied on the lingual side of the crown (close to the incisal edge) in order to evaluate the stress distribution ( s vM ) within the implant-abutment complex.
Results
The Beta values for groups SWT-EH (1.31), REG-EH (1.55), SWT-IH (1.83) and REG-IH (1.82) indicated that fatigue accelerated the failure of all groups. The higher levels of σ vM within the implant-abutment connection observed for platform-switched implants (groups SWT-EH and SWT-IH) were in agreement with the lower reliability observed for the external hex implants, but not for the internal hex implants. The reliability 90% confidence intervals (50,000 cycles at 300 N) were 0.53(0.33–0.70), 0.93(0.80–0.97), 0.99(0.93–0.99) and 0.99(0.99–1.00), for the SWT-EH, REG-EH, SWT-IH, and REH-IH, respectively.
Significance
The postulated hypothesis was partially accepted. The higher levels of stress observed within implant-abutment connection when reducing abutment diameter (cross-sectional area) resulted in lower reliability for external hex implants, but not for internal hex implants.
1
Introduction
In the first year after implant insertion and loading, early peri-implant bone loss commonly leads to a reduction in bone height, shown to vary as a function of quality and quantity of bone, implant and abutment designs, implant’s surface structure, insertion depths, arch region, and other factors . An attempt to hinder this process has resulted in the development of the platform switching concept, which consists in use of an abutment of smaller diameter connected to a implant of larger diameter. This connection shifts the perimeter of the implant-abutment junction inward towards the central axis (the middle of the implant), potentially improving the distribution of forces and placing the implant-abutment gap away from the peri-implant bone . It has been suggested that the inward shift of the implant-abutment gap may physically minimize the impact of the inflammatory cell infiltrate in the peri implant tissues, potentially reducing bone loss .
From a biomechanical perspective, previous in vitro studies have shown reduced levels of stress on peri-implant bone in platform-switched implants relative to matched implant-abutment diameters. Such potential for crestal bone level preservation has been shown in animal and clinical studies .
On the other hand, complications with implant-abutment connections is still a common clinical problem, especially in single-tooth replacements . When considering platform-switched implants, previous studies have shown an increased stress on the abutment and fixation screw, which may compromise the system biomechanical performance. Controversially, several published studies related to the mechanics of platform-switched implants have been restricted to analyzing the stress distribution on peri-implant bone and not on the overall system biomechanical behavior. To date, studies evaluating the mechanical behavior of platform-switched implants considering the stress distribution in implant-abutment complex are scarce and restricted to computer simulations , which do not consider several clinical variables (influence of fatigue damage accumulation and wet environment) previously reported as important factors to reproduce clinically observed failure modes .
Since the main challenges in the development of implant-abutment connection designs comprises reducing the incidence of mechanical failures while improving the interface between soft tissue and implant-abutment junction , the evaluation of reliability and failure modes supported by evaluation of stress distribution in each component of platform-switched connections may provide insight into the mechanical behavior of different configurations of implant-abutment connection. Therefore, the present study sought to assess the effect of abutment’s diameter shifting (regular and switched-platform) on reliability and failure modes of anatomically correct maxillary central incisor crowns varying the geometry of implant connection (internal and external hexagon). In order to evaluate the stress distribution within implant-abutment complex (implant, abutment and fixation screw), a three-dimensional finite element analysis was performed considering the variables. The postulated hypothesis was that platform-switched implants would result in increased stress concentration within the implant-abutment connection, leading to the systems’ lower reliability when subjected to step-stress accelerated life testing (SSALT).
2
Materials and methods
2.1
In vitro laboratory study: single load-to-fracture (SLF) and step-stress accelerated-life testing (SSALT)
Eighty-four commercially pure titanium grade 2 dental implants (SIN implants, São Paulo, SP, Brazil) were distributed in four groups ( n = 21 each) varying the abutment diameter (switched or regular platform) and the type of implant connection (internal or external hexagon) ( Fig. 1 and Table 1 ): (1) SWT-EH (switching platform and external hexagon implant); (2) REG-EH (regular platform and external hexagon implant); (3) SWT-IH (switching platform and internal hexagon implant); and (4) REG-IH (regular platform and internal hexagon implant).
Components | SWT-EH | REG-EH | SWT-IH | REG-IH |
---|---|---|---|---|
Implant | External hex (SUR 5011) | External hex (SUR 5011) | Internal hex (SIHS 5511) | Internal hex (SIHS 5511) |
5.0 mm diameter by 11.5 mm length | 5.0 mm diameter by 11.5 mm length | 5.0 mm diameter by 11.5 mm length | 5.0 mm diameter by 11.5 mm length | |
Ø prosthetic platform = 5.0 mm | Ø prosthetic platform = 5.0 mm | Ø prosthetic platform = 5.5 mm | Ø prosthetic platform = 5.5 mm | |
Abutment | Cemented (Al 4151) Ø platform = 4.1 mm | Cemented (Al 5051) Ø platform = 5.0 mm | Cemented (Al 4501) Ø platform = 4.5 mm | Cemented (Al 5501) Ø platform = 5.5 mm |
Ø neck’s region = 2.9 mm | Ø neck’s region = 2.9 mm | |||
Screw | fixation screw (PTQ2008) | fixation screw (PTQ2008) | fixation screw (PTQH16) | fixation screw (PTQH16) |
All implants were vertically embedded in acrylic resin (Orthoresin, Degudent, Mainz, Germany), poured in a 25-mm-diameter plastic tube, leaving the top platform in the same level of the potting surface ( Fig. 2 ). All groups were restored with standardized central incisor metallic crowns (CoCr metal alloy, Wirobond ® 280, BEGO, Bremen, Germany) cemented (Rely X Unicem, 3M ESPE, St. Paul, MN, USA) on the abutments, which presented identical height but different diameters ( Table 1 ).
For mechanical testing, the specimens were subjected to 30° off-axis loading ( Fig. 2 C). Three specimens of each group underwent single-load-to-fracture (SLF) testing at a cross-head speed of 1 mm/min in a universal testing machine (INSTRON 5666, Canton, MA, USA) with a flat tungsten carbide indenter applying the load on the lingual side of the crown, close to the incisal edge. Based upon the mean load to failure from SLF, three step-stress accelerated life-testing profiles were determined for the remaining 18 specimens of each group which were assigned to a mild ( n = 9), moderate ( n = 6), and aggressive ( n = 3) fatigue profiles (ratio 3:2:1, respectively) . Mild, moderate and aggressive profiles refer to the increasingly step-wise rapidness in which a specimen is fatigued to reach a certain level of load, meaning that specimens assigned to a mild profile will be cycled longer to reach the same load of a specimen assigned to either moderate or aggressive profiles. In the present study, the profiles started at a load that was approximately 30% of the mean value of SLF and ended at a load that was approximately 60% of the same value. The rationale for utilizing at least three profiles for this type of testing was based on the need to distribute failure across different step loads and allows better prediction statistics, narrowing confidence bounds. The prescribed fatigue method was step-stress accelerated life-testing (SSALT) under water at 9 Hz with a servo-all-electric system (TestResources 800L, Shakopee, MN, USA) where the indenter contacted the crown surface, applied the prescribed load within the step profile and lifted-off the crown surface. Thus, during SSALT each specimen was submitted to constant stress during a predetermined length of time. The stress on this specimen is thus increased step by step until failure (bending or fracture of the fixation screw and/or abutment) or survival (no failure occurred at the end of step-stress profiles, where maximum loads were up to 600 N) . Based upon the step-stress distribution of the failures, the fatigue data were analyzed using a power law relationship for damage accumulation and the use level probability Weibull curves (probability of failure vs. cycles) at a use stress load were determined for life expectancy calculations by using the software Alta Pro 7 (Reliasoft, Tucson, AZ) . The master Weibull curves obtained from the SSALT fatigue data were used to determine the reliability (the probability of an item functioning for a given amount of time without failure, 90% two-sided confidence bounds) of tested specimens for completion of a mission of 50,000 cycles at 210 N and 300 N load for group comparisons. For the mission reliability and β parameters calculated in the present study, the 90% confidence interval range were calculated as follows:
I C = E ( G ) ± Z α s q r t ( V a r ( G ) )
where CB is the confidence bound, E ( G ) is the mean estimated reliability for the mission calculated from Weibull statistics, Z α is the z value concerning the given CB level of significance, and Var ( G ) is the value calculated by the Fisher Information matrix .
Macro images of failed samples were taken with a digital camera (Nikon D-70s, Nikon, Tokyo, Japan) and utilized for failure mode classification and comparisons between groups. In order to identify fractographic markings and characterize failure origin and direction of crack propagation, the most representative failed samples of each group were inspected first under a polarized-light microscope (MZ-APO stereomicroscope, Carl Zeiss MicroImaging, Thornwood, NY, USA) and then by scanning electron microscopy (SEM) (Model S-3500N, Hitachi, Osaka, Japan) .
2.2
Three-dimensional finite element analysis (3D-FEA)
Four virtual 3D models were created using computer-aided design (CAD) software (SolidWorks 2010, Dassault Systèmes SolidWorks Corp., Concord, MA, USA) following design and dimensions observed in groups SWT-EH, REG-EH, SWT-IH and REG-IH. Each 3D CAD model represented all characteristics of the implant-abutment connection in order to reproduce the experimental conditions prevailing as a result of the mechanical tests ( Fig. 1 ). The components of the models consisted of a maxillary central incisor crown (Co–Cr alloy), a 50 μm-thick resin cement layer (Rely X Unicem), an abutment (titanium alloy), a fixation screw (titanium alloy), an implant (titanium alloy), and a cylinder created in the CAD software with the same dimensions of the plastic tubes used in the in vitro laboratory study ( Fig. 3 A ). The anatomically correct crown was generated from microcomputed tomography images in . dicom format (μCT40, Scanco Medical AG, Bruttisellen, Switzerland) and its cementation surface was designed to fit the abutments in all groups. The implant insertion hole in the cylinder (acrylic resin) was obtained by a Boolean subtraction ( Fig. 3 B).
The components were assembled, imported into FEA software (Ansys Workbench 12.0, Swanson Analysis Inc., Houston, PA, USA), meshed ( Fig. 3 C) (number of parabolic tetrahedral elements between 254,513 and 288,543; and number of nodes between 433,816 and 492,803) and tested for convergence prior to mechanical simulation. It was considered that the convergence criterion between meshes refinement was a change of less than 6% in the maximum simulated von Mises equivalent stress ( σ vM ) of the implant/abutment/screw components .
The FEA model assumptions were that: (1) all solids were homogeneous, isotropic and linearly elastic; (2) there were no slip conditions (perfect bonding) among components (set implant-abutment-screw, elastic modulus ( E ) = 110 GPa and Poisson’s ratio ( v ) = 0.35) ; (3) there was a uniform cement layer ( E = 8 GPa, v = 0.33) ; (4) there was a crown ( E = 220 GPa, v = 0.30) with similar dimensions (13 mm height with a mesiodistal width of 8.8 mm and buccal-lingual width of 7.1 mm) in all FEA models; (5) there were no flaws in any components; (6) the boundary conditions of the model were defined on the bottom and lateral surface of the cylinder of acrylic resin ( E = 1.37 GPa, v = 0.30) 1
1 Manufacturer’s information.
to represent the constrained of x , y and z directions (displacement = 0) ( Fig. 3 B). As in the mechanical tests, one 30° off-axis load (300 N) was applied on the lingual side of the crown, close to the incisal edge ( Fig. 3 B). Regions of higher von Mises equivalent stress ( σ vM ) were determined within implant-abutment connection for all models.
2
Materials and methods
2.1
In vitro laboratory study: single load-to-fracture (SLF) and step-stress accelerated-life testing (SSALT)
Eighty-four commercially pure titanium grade 2 dental implants (SIN implants, São Paulo, SP, Brazil) were distributed in four groups ( n = 21 each) varying the abutment diameter (switched or regular platform) and the type of implant connection (internal or external hexagon) ( Fig. 1 and Table 1 ): (1) SWT-EH (switching platform and external hexagon implant); (2) REG-EH (regular platform and external hexagon implant); (3) SWT-IH (switching platform and internal hexagon implant); and (4) REG-IH (regular platform and internal hexagon implant).
Components | SWT-EH | REG-EH | SWT-IH | REG-IH |
---|---|---|---|---|
Implant | External hex (SUR 5011) | External hex (SUR 5011) | Internal hex (SIHS 5511) | Internal hex (SIHS 5511) |
5.0 mm diameter by 11.5 mm length | 5.0 mm diameter by 11.5 mm length | 5.0 mm diameter by 11.5 mm length | 5.0 mm diameter by 11.5 mm length | |
Ø prosthetic platform = 5.0 mm | Ø prosthetic platform = 5.0 mm | Ø prosthetic platform = 5.5 mm | Ø prosthetic platform = 5.5 mm | |
Abutment | Cemented (Al 4151) Ø platform = 4.1 mm | Cemented (Al 5051) Ø platform = 5.0 mm | Cemented (Al 4501) Ø platform = 4.5 mm | Cemented (Al 5501) Ø platform = 5.5 mm |
Ø neck’s region = 2.9 mm | Ø neck’s region = 2.9 mm | |||
Screw | fixation screw (PTQ2008) | fixation screw (PTQ2008) | fixation screw (PTQH16) | fixation screw (PTQH16) |
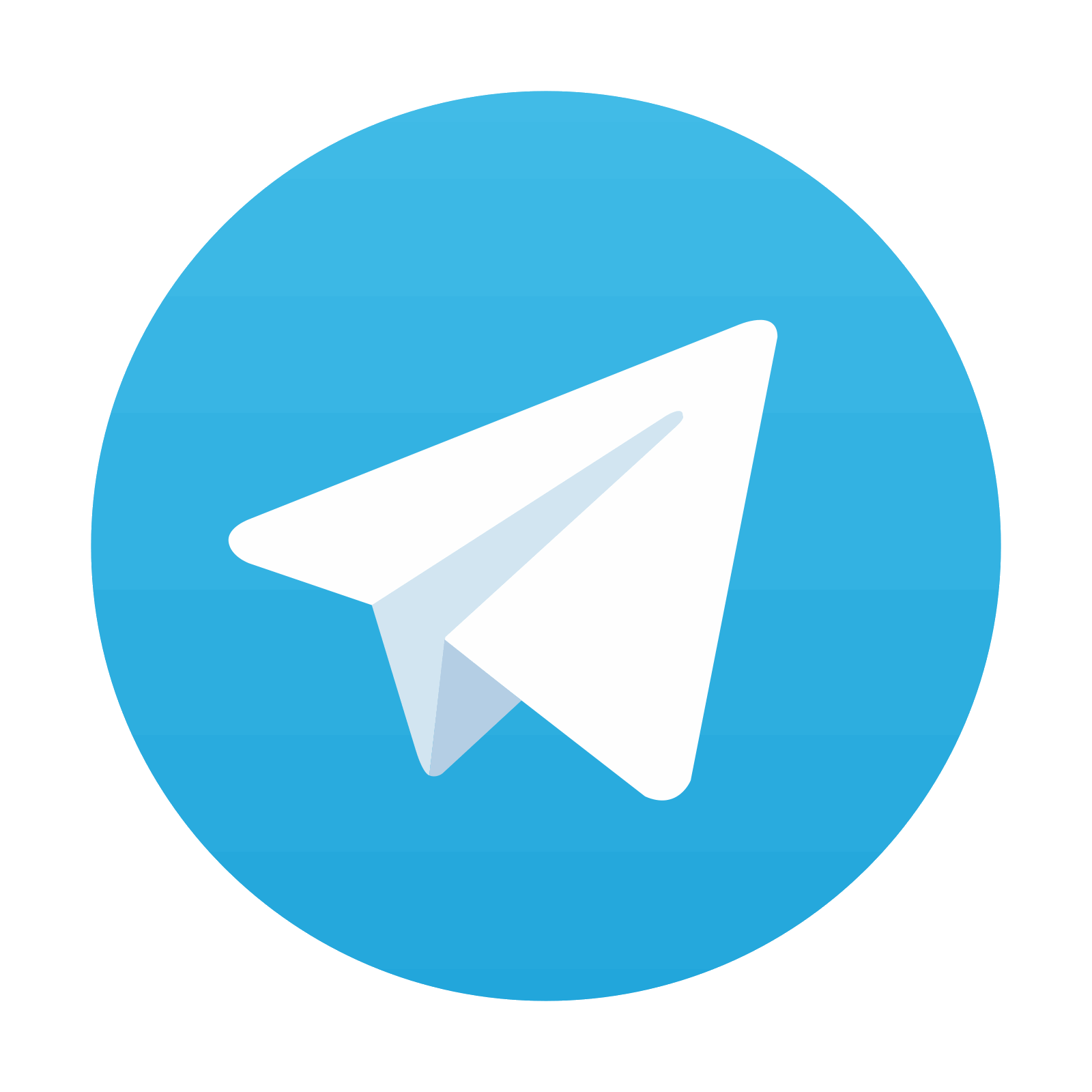
Stay updated, free dental videos. Join our Telegram channel

VIDEdental - Online dental courses
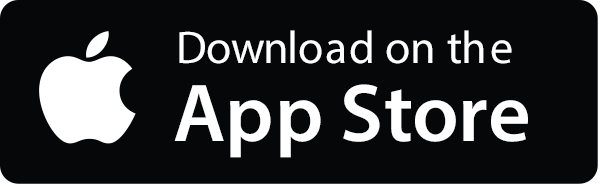
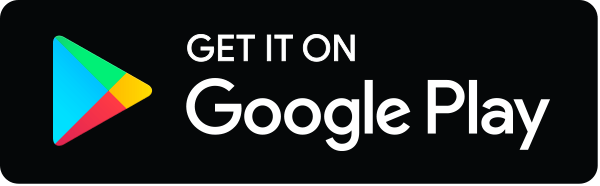
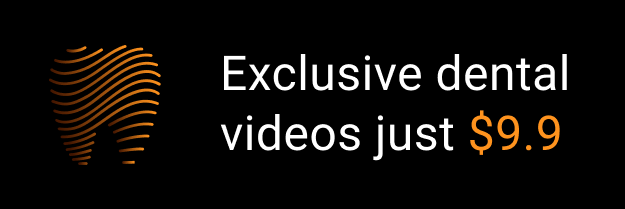