1
Biology of bone regeneration in augmentative procedures
1.1 Introduction
Regenerative dentistry critically depends on the functional understanding of bone biology – to be precise, bone development, bone modeling and remodeling and bone regeneration – in a physiologic but also in a pathologic and pharmacologic context. Bone biology also describes the cellular and molecular regulation behind Wolff’s law (form follows function), which was later refined by Frost’s Mechanostat theory.44 Bone biology is a molecular and cellular system that is essential for mammalian evolution. Besides being a framework connecting to tendons and muscles and for protecting the bone marrow, the skeleton is a storage for calcium and phosphate that is transported via the umbilical vein and later through the mother’s milk into the fetus and newborn. Understanding the delicate interplay of bone-forming cells and bone-resorbing cells – which act in concert with the osteocyte located within the bone matrix, the blood vessels providing support for the respective progenitors, and the cells originally dedicated to the immune system – provides one part of the information necessary for progress in medicine.
The concert has to be orchestrated, which is, in the context of bone biology, the cell-to-cell communication involving the classical path. This path can roughly be divided into local and systemic regulation. Local regulation includes cell communication via cytoplamatic connections or the release of signaling molecules, with particular receptors on the respective target cells. Systemic regulation refers to the endocrine system, whereby hormones or growth factors are released and transported via the bloodstream to target cells elsewhere in the body. It is fascinating to imagine all the different levels – molecular, cellular, tissue, and organ – to be coordinated, with the same aim of homeostasis. In a broader sense, not only does homeostasis maintain the tissue (which would be bone remodeling), it is also the mechanism to regain homeostasis after injury, thus bone regeneration. However, the delicate cellular and molecular mechanisms aiming for homeostasis are sensitive to change; for instance, the drop of steroid hormones during menopause, which causes not only enhanced but also disbalanced bone remodeling and ultimately leads to bone loss and postmenopausal osteoporosis. The mechanical integrity, particularly of the trabecular bone, is rapidly impaired, and fragility fractures of the vertebra and the hip become clinical hallmarks of the disease.107 Postmenopausal osteoporosis is but one example of how bone homeostasis undergoes a catabolic shift that, together with age-related changes, leads to a progression of bone loss over time.
The main focus of this chapter, however, is to provide an explanation of autograft consolidation, and to discuss the clinical success of this therapy at the molecular and cellular levels. With an emphasis on bone augmentation, the chapter is intended to supplement the essential information on bone regeneration that has been obtained from histologic and biomechanical analyses.
It is a well-accepted fact that osteoblasts form the bone40 and osteoclasts resorb it.12,121 The osteocytes are important in that they are the masters of regulation in bone remodeling.33 The blood vessels are also important as they serve as a source of renewal and, in particular, as a transport medium for the precursor cells of osteoblasts and osteoclasts;78,134 they are also key in terms of inflammation, and are therefore relevant in pathologic conditions such as inflammatory osteolysis.55,84 In this context, classical questions are addressed in the chapter, such as the evidence that autografts are considered “osteoconductive, osteogenic and osteoinductive,”98 and the possible mechanisms of graft resorption.
1.2 Cells of bone remodeling
Three cell types are characteristic of bone tissue and are responsible for bone formation, maintenance, remodeling, and repair. However, bone biology and bone metabolism comprise a complexity of interactions involving many factors, including growth proteins and many humeral messages and events that are not described in this chapter. One main goal of the chapter is to provide an update on the essential activity of the bone-forming cells (osteoblasts) and the bone-resorbing cells (osteoclasts), with special attention paid to the osteocytes and their important role in the maintenance of bone structure.
1.2.1 Osteoblasts
These cells originate from pluripotent mesenchymal stem cells through the activation of a series of transcription factors62 partially involving members of the bone morphogenetic protein superfamily.81,99 Osteoblasts are present in layers on the bone surface. In all active bone-formation sites, they are responsible for extracellular matrix production (osteoid) and subsequent mineralization. Osteoblasts are polarized cells with a mineral-facing side through which the matrix is extruded. Once osteoid production stops, some osteoblasts are trapped in the extracellular matrix and differentiate into osteocytes, which are located in the bone lacunae. On the one hand, neurocranial bones,21 including the mandible (except the mandibular condyle) and maxilla as well as part of the clavicle, are formed by membranous ossification. This is a direct ossification without a cartilaginous phase, where differentiated osteoblasts lead to osseous matrix formation through mesodermal and ectomesodermal cellular condensation. On the other hand, the appendicular and axial skeleton follows an endochondral ossification route. A temporary cartilaginous scaffold is produced by chondrocytes, which mature and hypertrophy in a second stage. In a third stage, this cartilaginous matrix becomes mineralized. Finally, a vascularization is established that allows, at first, the arrival of osteoclasts (or chondroclasts), which lead to the resorption of the calcified cartilaginous matrix and, following that, the differentiation of osteoblasts that will replace the cartilaginous scaffold by a bony matrix. This matrix will lead to the formation of the trabecular structure of the long bones.91
Osteoblasts can produce three types of bone: woven bone, primary parallel-fibered bone, and lamellar bone. The difference between these bone types is related to the orientation of the collagen fibrils: In woven bone, the fibrils are three-dimensionally and randomly distributed due to the rapidity of osteoid deposition and mineralization (Fig 1-1). Compared with mature lamellar bone, this bone is more elastic and mechanically less consistent due to the low level of mineralization and the lack of a specific orientation of the collagen fibers. In adults, this type of bone is produced during healing processes, and it is the only bone able to grow in the absence of a pre-existing mineralized tissue. Woven bone forms ridges and roots between and around the blood vessels (Fig 1-2). Primary parallel-fibered bone is characterized by a more parallel distribution of the collagen fibrils, and is typically produced during periosteal and endosteal bone apposition. The mechanical properties are as weak as those of woven bone. Lamellar bone is a well-organized mineralized tissue. Collagen fibrils are distributed in parallel layers that have a thickness of 3 to 5 µm. Osteoid production is slow (1 to 2 µm per day) compared with woven bone, and it takes about 10 days to be mineralized at a well-defined mineralization front. Lamellar bone needs a pre-existing bone surface to be produced by osteoblasts, which means that, unlike woven bone, it is not able to bridge gaps.
Fig 1-1 Osteoblasts produce bone on the surface of a host bone. Bone formation occurs on the surface of existing bone (pink). New bone (dark purple) is lined by seams of osteoblasts and arranged in osteonal structures. Osteoid (barely stained) is bone that is not yet mineralized. The direction of new bone formation can be anticipated by the sprouting of extension into the defect area. [The image is of pig bone.]
Fig 2-1 Osteoblast seams during the early stages of bone formation. Bone formation is the consequence of osteoblast activity. Osteoblasts dominate the scene, and non-mineralized bone (osteoid) is visible. [The image is of pig bone.]
When not active in osteoid production, osteoblasts can differentiate into bone-lining cells. This particular conformation determines a flat distribution of osteoblasts over the bone surface, creating a barrier-like layer between the bone and the extracellular space that seems to be responsible for ion exchange. The bone lining cells may also be responsible for bone resorption through two mechanisms: the first is determined by cell contraction and subsequent bone surface exposition; the second is defined by the direct secretion of osteoclast activating factors.
1.2.2 Osteocytes
Osteocytes are characterized by a slower metabolism than osteoblasts and present elongations of the cytoplasmic membrane that connect osteocytes to each other and to the surface cells through gap junctions, creating a three-dimensional canalicular network in the mineralized tissue that is particularly impressive in the osteons (Fig 1-3). The diffusion of nutrients and ions, otherwise impossible, is guaranteed by this cell network. A limit in diffusion through the canalicular system exists, which is approximately 100 µm. This is also the mean wall thickness of osteons in the cortical bone and also the packets in trabecular bone. The osteocytes, which control the effector cells (the osteoclasts and osteoblasts),7,10,33 require a long lifespan because they are embedded in lacunae within the mineralized matrix, and are connected via dendritic processes that run through the canaliculi. The dense, interconnected network that spans the entire skeleton also connects to blood vessels and to the cells on the bone surface, e.g. the lining cells, osteoblasts, and osteoclasts. As recently summarized,15 1 mm3 of bone contains about 20,000 to 30,000 osteocytes, each having 100 dendritic processes and a radius of approximately 70 nm. Around 40 billion (109) osteocytes with 20 trillion (1012) connections and a total length of dendritic processes of 200,000 km can be calculated for the entire skeleton. The surface area and the volume of the lacuno-canalicular network are around 200 m2 and 40 cm3, respectively. Osteocytes are not only interconnected via their dendritic processes but are surrounded by a liquid that connects them to the overall circulation. Osteocytes are obviously predestined to control bone homeostasis at the local and systemic levels. For example, osteocytes are the cells that almost exclusively produce sclerostin, an inhibitor of the Wingless-related integration site (Wnt) signaling pathway.129,130 The molecular function becomes obvious when one considers bone overgrowth, including the jaw and facial bones of sclerosteosis and van Buchem disease, which are caused by the loss of sclerostin expression and secretion, respectively.128,130 Mouse models lacking sclerostin also display systemic high bone mass, and increased alveolar bone and cementum.77,82 Osteocytes are also a main source of RANKL required for physiologic bone remodeling and in pathologic situations, including ovariectomy,45,94 secondary hyperparathyroidism140 or glucocorticoid excess.92 Mice lacking osteocyte-derived RANKL even resist the bone loss caused by tail suspension.93 Recently, osteocyte-derived RANKL was considered relevant in inflammatory osteolysis51 and orthodontic tooth movement.109 Thus, osteocytes control bone formation and bone resorption during good health and during disease, including their expression of sclerostin and RANKL.
Fig 3-1 Osteoclasts (boneresorbing cells), osteoblasts (bone-producing cells), and osteocytes. Osteoclasts are multinucleated cells that are exclusively capable of resorbing bone. In this image, which is a detail taken from Fig 1-7, a group of osteoclasts is resorbing bone next to a seam of osteoblasts, which are producing new bone. An osteoid seam is visible below the osteoblasts. Osteocytes are embedded in the bone. [The image is of pig bone.]
1.2.3 Osteoclasts
Osteoclasts and osteoblasts are partners in the bone remodeling process – osteoblasts are the bone-building and osteoclasts the bone-resorbing cells (Fig 1-4a and b). Osteoclasts are therefore specialized in the breakdown of calcified tissue. Hematopoietic cells, particularly those of the monocyte lineage, are the pool of progenitors that have the potential to become osteoclasts; otherwise, they develop into macrophages or dendritic cells with a focus on the immune system. The molecular signature to drive osteoclastogenesis was discovered almost two decades ago, with the introduction of the RANKL-OPG system, the agonist, and the respective antagonist.23,61,118 Mouse models that lack RANKL73 or the respective receptor RANK38 develop severe osteopetrosis, indicated by the lack of a bone-marrow cavity and non-disrupted teeth. In contrast, mice lacking RANKL-OPG acquire a fulminant osteoporosis.14,111 RANKL was considered the ‘bottleneck’ of osteoclastogenesis. Mature osteoclasts are characterized by the sealing zone that sticks the osteoclasts to the mineralized bone surface, surrounding that extensively folded ‘ruffled border,’ where the protons (to lower the pH) and the proteases (to digest the collagen, mainly cathepsin K) are transported into the space facing the naked bone matrix.121 Osteoclasts are considered to be of “great beauty”18 and are not simply “bone eaters”27 as they contribute to bone formation and also interact with the hematopoietic system, including the stem cell niche and adaptive immune cells.
Fig 1-4 Creation of osteons by basic multicellular compartments (BMU). The BMU defines the site of bone remodeling. (a) Tunneling of cortical bone by multinucleated osteoclasts. (b) This image is characteristic for the activity of bone forming osteoblasts with an osteoid layer, rebuilding the concentric structure of osteons. [The image is of pig bone.]
The main physiologic function of osteoclasts is to participate in bone remodeling. Localized in Howship’s lacunae, which represent the active resorption sites on a bone surface, osteoclasts are indicated as multinucleated cells staining positive for tartrate-resistant acid phosphatase. The acidophil cytoplasm contains vacuoles, which indicate resorption. In trabecular bone, osteoclast resorption does not usually exceed 70 µm before a team of osteoblasts fills the space with new bone. Howship’s lacunae are part of the bone remodeling compartment (BRC) canopy.35 In cortical bone, however, the basic multicellular unit (BMU) defines the site of bone remodeling.106 Here, osteoclasts produce a tunnel in the cortical bone that is closed in concentric layers of new bone by the bone-forming osteoblasts with a blood vessel in the center, culminating in the characteristic histologic picture of the osteons in a transversal section (Fig 1-5). Even though the two remodeling compartments are not identical in structure, there is the common principle of the coupling: when osteoclastic bone resorption has ceased, osteoblastic bone formation is initiated. Preosteoclasts are not only important for bone renewal and remodeling but also for bone revascularization,137 thereby possibly supporting the sprouting of blood vessels at the site of bone regeneration.
Fig 1-5 Osteon with osteocytes being connected via their canaliculi. The osteon is a functional bone unit consisting of a central canal filled with soft tissue, with bone lamellae arranged concentrically around it. They can be found in the substantia compacta of the bone. Osteocytes are interconnected via canaliculi. They are in contact via canaliculi with the lining cells in the central channel. [The image is of human bone, from an implant extraction.]
1.3 Biology of bone regeneration
Bone regeneration is another important aspect of bone biology. Bone regeneration works perfectly in the sense that no scar tissue is formed, which contrasts with the classical skin wound healing in adults, where the defect is left with a matrix rich in collagen but poor in cells. This is summarized in excellent reviews on bone regeneration, particularly in fracture healing30,42 and wound healing.90,113,143 Both events start with the formation of a blood clot, where the coagulation cascade of proteases culminates in the formation of thrombin, which cleaves fibrinogen. The fibrin itself assembles into a transient extracellular matrix, where platelets are activated and form aggregates, together with erythrocytes. Growth factors and other molecules are released, attracting neutrophils into the blood clot to clean the defect site. Macrophages appear later in the blood clot. To make space for the granulation tissue, which is characterized by the sprouting of blood capillaries into the new tissue and the concomitant appearance of fibroblastic cells, fibrinolysis is initiated. The invading cells release activators for plasminogen being stored in the blood clot – it is plasmin that cleaves the fibrin matrix. Interestingly, mouse models lacking fibrinogen allow bone regeneration,141 while those lacking plasminogen show impaired bone regeneration.64 These findings highlight the importance of fibrinolysis over the formation of the fibrin matrix.
Mouse models have also helped in the understanding of the importance of macrophages in bone regeneration, as they were shown to be in wound healing, early on. The depletion of macrophages and the genetic modification of the cells to erase their activity culminate in impaired bone regeneration, including intramembranous ossification, which is the more relevant path in regenerative dentistry compared with the endochondral ossification that is typically observed in fracture healing.95,135 However, the role of macrophages is not restricted to a defect situation. For example, macrophages form a canopy structure over mature osteoblasts during bone remodeling, suggesting that they interact via juxtacrine and a paracrine mechanism that remains to be fully elucidated.25 The clinical implication of this fundamental principle in regenerative dentistry is unclear, but it opens a wide arena for research that may involve biomaterials. Mouse models have also provided evidence that at least a transient inflammation is required for bone regeneration, as, for example, the knockout of TNFα24,48 and COX-2142 caused impaired bone regeneration. Moreover, in bones lacking bone morphogenetic protein 2 (BMP-2), the earliest steps of fracture healing seem to be blocked,125 and it is possible that the local inflammation controls the expression of BMP-2, at least in vitro.46 To what extent macrophages are involved in the inflammation required for bone regeneration has not yet been investigated. Also, here, the clinical relevance of these observations should be interpreted with care. For example, painkillers should not be a great concern in regenerative dentistry as they do not completely block cyclooxygenases and are only used temporarily.49 Bone regeneration is not influenced or jeopardized when inhibitors of TNFα are used,122 as in a situation of chronic inflammation, including rheumatoid arthritis and colitis ulcers. Thus, findings from the extreme situation of a gene knockout or enhanced expression in mouse models should be interpreted carefully in the clinical context.
Mouse models also support the role of BMP-2 during bone regeneration.125 Molecular screening approaches have revealed a long list of growth and differentiation factors that are differentially expressed during bone regeneration, in particular fracture healing, that play a major role in bone formation.54 For example, BMP-4126 and BMP-7127 have no effect on fracture healing, but Wnt signaling is crucial for bone regeneration, based on observing with a sclerostin antibody and sclerostin knockout models.4 The Hedgehog signaling pathway also plays a critical role in osteoblasts during fracture repair.6 While it is obviously the orchestrated interplay of a large spectrum of local and systemic signals that drives osteoblastogenesis, and thus bone regeneration, there are growth factors such as BMP-2 that are not only supportive but also essential for proper bone regeneration, and thus likely also for graft consolidation. However, considering the complex interplay of immune cells, endothelial cells, osteocytes, and osteoclasts in controlling bone formation, many molecular mechanisms remain to be discovered.
Histology has provided insights into the defect sites, showing that osteoclasts are already active a few days after the injury, and that bone formation by osteoblasts is clearly visible 10 days after implant insertion in a pig model.131 The new bone grows fairly rapidly, at approximately 10 µm per day, and sprouts into the defect area. Then, lamellar bone is formed on the surface of the woven bone, which overall is independent of osteoclasts and is thus strictly in an anabolic phase until bone remodeling is initiated. Finally, the woven bone and the primary lamellar bone are replaced by secondary lamellar bone, which is the final stage of bone regeneration, and bone remolding takes over. What histology convincingly demonstrates is that the new bone grows into an area rich in blood vessels, but without touching them.131 Considering the three choices of osteoblasts – to become an osteocyte, to become a lining cell, or to die – a supply of new osteoblasts to drive bone regeneration seems mandatory. The close proximity of osteoblasts has always pointed toward blood vessels as the source of the mesenchymal progenitor cells, but evidence was scarce. Today, advanced mouse models have supported this hypothesis, e.g. by showing that only a certain type of endothelial cells (H-type) is associated with osteogenic precursors, which resemble pericytes but are perhaps a distinct population.78,134 Blood vessels in the growing long bones are rich in osteogenic precursors, and are predetermined as they express the differentiating marker osterix.78 Blood vessels in the bone marrow, however, do not carry this cell population. It is reasonable to suggest, under the premise that (to some extent) bone regeneration recaptures bone development, that these osteogenic blood vessels also sprout into the defects after implant insertion or bone augmentation. Moreover, Prx1-Cre mouse models support the role of the periosteum as a rich source of osteogenic cells. These cells can efficiently contribute to cartilage and bone formation upon injury.41 This knowledge now has to be translated into higher animals and its clinical relevance determined.
Fig 1-6 Dental implant after 5 days of osseointegration in a pig jaw, from a study by Vasak et al.131 Five days after implant placement, close to the implant surface, the bone is fragmented, squeezed, and heat damaged (dark pink). Osteocytes in the vicinity are dead and dying. Osteoclasts (white asterisks) are digging bone channels, sprouting from existing bone canals (osteons) to reach and resorb the damaged bone. They are about to reach the most damaged bone close to the implant and will soon remove it.
If the overall hypothesis is correct, the formation of this subtype of endothelial cells carrying the osteogenic cells is essential for bone regeneration, and thus also for regenerative dentistry. However, since the pioneer findings with parabiosis experiments,22 there is good evidence that blood vessels provide the progenitors of osteoclasts. Here, the bone marrow is irradiated and thus osteoclastogenesis is impaired; however, it is regained when the circulation is connected to a vital mouse, so that osteoclast progenitors have to be carried via the bloodstream. Taken together, the blood vessels are key for osteoblastogenesis and osteoclastogenesis – and consequently also for bone regeneration.
1.3.1 Osseointegration of dental implants
It is known from preclinical histologic investigations in minipig131 and mandibular canines,9 but also by measuring implant stability in a clinical setting,108,132 that within the first week, resorption of the peri-implant bone dominates the scene, before bone formation takes over (Fig 1-6). This early catabolic process is required for the removal of micro-damaged necrotic bone, which is characterized by dying osteocytes. After around 1 week, the osteoclasts have disappeared, leaving behind an osteophilic surface onto which new bone is deposited (Fig 1-7).9,131 Small defects, as they occur between the local bone and the threads of the implants, are bridged with new bone. These relatively small distances are known as jumping distances.11 Primary woven bone formation shows a typical picture, with blood vessels in the center of an open ring of new bone (Fig 1-8). This image supports the assumption that the origin of the cells required for bone formation is based on pericytic progenitor cells of sprouting blood vessels.78,134 This bone is immature (woven bone).9 Subsequently, lamellar bone will strengthen the woven bone that later undergoes modeling and remodeling.
Fig 1-7 Dental implant after 10 days of osseointegration in a pig jaw, from a study by Vasak et al.131 Ten days after implant placement, feeble trabeculae of new bone have already replaced the damaged old bone. New bone continues to grow. Batches of osteoclasts are resorbing the remaining damaged bone.
Modeling refers to the functional adjustments based on the reaction of the bone to biomechanical stimuli according to Wolff’s law and Frost’s Mechanostat theory.44 We are beginning to understand today how resident bone cells perceive and translate mechanical energy into biologic signals. These signals transiently uncouple the remodeling equilibrium of osteoblasts and osteoclasts; otherwise, no structural change of bone anatomy is possible.96 Remodeling, then, ensures the preservation of bone quality and long-term implant success. According to current hypotheses, the necrotic bone areas created during loading are resorbed by osteoclasts and are immediately replaced by osteoblasts, which was originally postulated by Frost44 and has now been proven to involve the apoptotic and necrotic death of osteocytes.65,66 Osseointegration is therefore not only the transition from mechanical primary instability to biologic secondary stability due to bone regeneration;86 it also requires the continual maintenance of bone quality through remodeling. Bone regeneration and bone remodeling are not necessarily subjected to the same regulatory mechanisms; for example, bone formation during early fracture healing can take place without the resorptive activity of osteoclasts,50,85 while bone remodeling is strictly based on the coupled effect of osteoclasts and osteoblasts.112 What is true for osseointegration is also observed during graft consolidation – the osseointegration of autografts.
Fig 1-8 Dental implant after 10 days of osseointegration in a pig jaw, from a study by Vasak et al.131 Dynamics of early bone formation in the grooves of an implant. New bone (purple) is growing on the implant surface as well as on fragments of old bone that is not resorbed (light pink). Erythrocytes (dark blue) indicate the presence of blood vessels.
1.3.2 Autogenous bone grafts
Even though there is a long tradition of considering autologous bone a gold standard, this claim needs to be specified. Bone transplantation dates back to 1879, when a bone allograft was performed on a 3-year-old boy affected by a huge humeral bone loss.36 Reviews such as the one by Albee in 19302 are worth reading from an orthopedic perspective.2 From back in the pioneer days to today, the primary intention of bone grafting was to allow the replacement of missing bone in defects of critical size.103 In implant dentistry, starting in the 1980s, bone grafts harvested from the ilium and the mandible were used to reverse alveolar atrophy of the maxilla and mandible.124 At that time, bone regeneration with autografts was also being compared to those filled with bone substitutes.
Preclinical research in pig mandibular defects convincingly showed that, after 2 weeks, almost twice as much new bone formation had occurred in the presence of autologous bone chips compared with bone substitutes;16,59,60 however, this does not necessarily mean that autograft bone chips support bone formation. In the same model, when defects were foiled with corticocancellous blocks particulated by a bone mill, bone scraper, piezosurgery, and bone slurry, bone formation at 1 week was restricted to the borders of the defect, making a total of 3% to 4% of new bone; no bone formation was observed in the center of the defect.100 Bone chips that filled around 20% to 30% of the area were significantly covered by osteoclasts.100 After 2 weeks, bone formation had increased, covering 20% to 30% of the defect area, while within only 1 week, 20% to 40% of the bone chips were resorbed.100 This dynamic phase of graft resorption followed by extensive bone formation continues after 4 and 8 weeks, albeit slowing down overall. The low resorption and the favorable osteogenic potential of autographs is supported by the research of the editor of this book.
1.3.2.1 Bony lid technique
Already in 1987, Khoury reported his clinical data on the bony lid technique for the apical root resection of mandibular molars.71 In another study, he reported his prospective data on this technique in pre-implant and implant surgery.67 The bony lid is a cortical bone plate obtained by the cutting and luxation of parts of the mandible (see Chapter 4). The cortical bony lid can also be split into two halves that are used as a bony sheet (stabilized by micro screws) that holds the bone particles in place, thereby molding the augmentation and implantation site (see Chapter 4). At the re-entry 3 months later, the average width of the alveolar crest after placing the bony lid showed a loss of only 0.5 mm, which is around 7% of the original dimension, suggesting good volume stability.
1.3.2.2 Split bone block (SBB) technique
Based on the principles of the bony lid technique, Khoury went on to harvest monocortical bone blocks with the MicroSaw, especially from the retromolar area.69 The bone blocks were longitudinally split and thinned with a bone scraper, gaining at the same time a significant quantity of autogenous bone chips. The thin bone blocks were then stabilized at a distance from the alveolar crest with micro screws to recreate alveolar ridges with sufficient volume and thickness, especially for vertical bone augmentation, and allowing for later implant placement in the prosthetically required position. The space between the thin bone blocks and the remaining alveolar crest was filled with the scraped autogenous bone chips. After 3 months, the implants were inserted into the grafted area70,72 (see Chapter 4). After 3 months of healing, the grafted area was exposed, and the height and width of the grafted area measured. At the same time, bone cores from the planned implant site were removed for histology and histomorphometry using trephine burs (Fig 1-9). The mean bone resorption was 3.9% in the vertical and 7.2% in the horizontal dimension at the time of implant insertion in the case of 3D vertical augmentation in the posterior maxilla. After 10 years of observation, the mean vertical bone resorption measured on the radiographs was 8.3%. The core biopsies obtained prior to implant placement in the two-stage approach show larger (Fig 1-10a to c) and smaller (Fig 1-11a to c) bone chips, which now are integrated into the new bone. Noticeably, the bone surface is not occupied by multinucleated cells, and new bone formation is obvious, making the augmented area ideal for supporting the process of osseointegration of dental implants. This explains the long-term stability of the vertical grafted area with the osseointegrated implants.
Fig 1-9 Biopsy from the split bone block (SBB) technique. The space between the thin bone blocks and the remaining alveolar crest was filled with the scraped autogenous bone chips. After 3 months, the implants were inserted in the grafted area.70,72 After 3 months of healing, bone cores from the planned implant site were removed for histology. In this image, the new bone is stained purple, while the old pristine bone and the transplanted bone chips are pink.
Fig 1-10a to c New bone formation on the surface of transplanted bone chips. In the SBB technique, scraped autogenous bone chips fill the space between the cortical bone blocks. After 3 months, healing bone cores from the future implant site were removed. The new bone is stained purple, while the transplanted bone chips are pink. Note the cement lines of the transplanted bone, which are signs of previous bone remodeling. Osteocyte lacunae are either empty or filled.
Fig 1-11a to c New bone formation on the surface of transplanted bone chips. Scraped bone chips can have various shapes and may even resemble bone dust. In the SBB technique, after 3 months of healing, the bone chips are covered by new bone and no obvious signs of resorption are visible.
1.3.2.3 Bone core technique
For small augmentations, the bone core technique is recommended. A bone core is harvested using trephine burs of different diameters, but on average a 3.5-mm external and a 2.5-mm internal diameter (see Chapter 4). The bone cores are used together with bone chips to augment the bone immediately after implant placement. This trabecular bone core can be used analogous to the cortical bone plate, providing a small bony sheet for the bone particles, again requiring stabilization with micro screws. After 3 months of healing, the implants and the grafted bone are exposed, and the width of the grafted area measured. Bone cores grafted completely inside the bony contours demonstrated no resorption 3 months postoperatively, while in most cases bone cores grafted partially outside the bony contours showed partial resorption of the bone outside the bony contours.67 Similar to the bony lid technique, at the re-entry 3 months later the average width of the area reconstructed with the trabecular bone core only lost 0.3 mm, which is around 13% of the original dimension, again suggesting good volume stability. What we can learn from this approach is that, in a clinical scenario, the resorption of cortical bone plates as well as trabecular bone cores is low, and that bone chips are well integrated into the newly formed bone after 3 months.67–69 Taken together, autografts in this particular indication allow and may even support the occurrence of natural bone formation originating from the host bone and maybe also from the transplanted autografts. In addition, and interestingly, the augmented volume remains rather stable, with around 7% to 13% resorption after 3 months.
Bone resorption also occurs after tooth extraction, as reported in canine models5 and clinical cases,104 and when the facial bony wall is thin it even disappears, probably due to the lack of vascular supply.26 The questions are as exciting as they are important: 1) Why are transplanted autographs resorbed? 2) Why does this resorption occur partially but not completely, depending on the size and anatomy of the autograft? 3) Why is it difficult to predict the extent of resorption?
There are certainly many reasons for bone resorption – some are known (e.g. the influence of muscle activity), while others are as yet unknown. In case of human sinus augmentation with pure autogenous grafts, around 40% of bone volume is lost within 6 months, probably through the respiratory pressure on the sinus mucosa covering the grafted and non-mechanical resistant trabecular bone,31,47,101 similar to a canine model.102 In alveolar cleft patients, transplanted iliac bone showed comparable bone resorption rates of less than 40% within 6 months.39,136 On a cellular level, the resorption of autologous bone chips by osteoclasts within 1 week is particularly obvious in the pig mandibular defect mentioned above.100 It seems that the resorption of transplanted bone that undergoes necrosis is prone to resorption – similar to local bone areas, with microcracks that undergo fatigue damage and are replaced by remodeling.107
1.4 Autograft resorption
There must be at least one mechanism that controls the resorption of the transplanted bone. One possible explanation could be the function of osteocytes, which are ubiquitously present in the bone, forming a coherent network.15 Osteocytes can control the formation of boneresorbing cells by expressing RANKL,89,138,139 a central agonist of osteoclastogenesis.118 In addition, there is increasing evidence from mouse research that dying osteocytes significantly promote osteoclastogenesis.120 The resorption of alveolar bone upon tooth extraction, implant placement, and early stages of graft consolidation after bone transplantation may also be associated with osteocytes. In all cases, the bone tissue is separated from the blood vessels, and therefore the oxygen and nutritional supply of the osteocytes by passive diffusion is limited or even impossible. Consequently, osteocytes die, and, by a molecular mechanism, promote the expression of RANKL by the adjacent osteocytes that, in turn, can initiate osteoclastogenesis.65,66 Molecules released by dying osteocytes can also increase the sensitivity of osteoclast progenitors to RANKL via a C-type lectin receptor. Importantly, unloading-induced bone loss also requires the dying osteocytes to enhance bone resorption via their expression of RANKL.17 Accordingly, bone resorption, presumably also in dentistry, is bound to dying osteocytes and does not progress uncontrolled. However, it is reasonable to suggest that dying osteocytes can not only transiently push osteoclastogenic resorption, but also the reparation process, through new bone formation as a normal physiologic reaction of remodeling. Thus, the initial boost of bone resorption that occurs at implant sites131 and upon bone grafting100 is followed by the attraction of osteogenic progenitor cells that become bone-forming osteoblasts on the surface of the host bone, the autografts, and also on biomaterials, including dental implants.131
Elegant preclinical studies in mouse models support the hypothesis through experiments in which the apoptosis of the osteocytes were analyzed after the preparation of an implant bed, e.g. drilling tools create a zone of dead and dying osteocytes around the osteotomy29 that is increased as a function of the insertion torque.19 The pharmacologic suppression of apoptosis can also reduce bone atrophy upon extraction in a rat model.105 Thus, the strategy exists to develop low-invasive drill designs that initiate low heat and mechanical friction, with the overall goal of preserving osteocyte viability.1,28 In a bovine femora, test drills can reach 47°C, particularly after repeated use,20 which is similar to experiments performed with polyurethane foam blocks,43 a temperature that causes osteocyte damage and RANKL expression in a rat model.37 Cutting energy is converted into heat.80 Bone chips produced by drilling80 presumably follow the dying osteocytes – RANKL expression axis and are removed by osteoclasts before the osteoconductive properties come into play. It therefore seems relevant to pay special attention to atraumatic procedures when placing implants, extracting teeth, and probably also removing bone grafts, with the overall aim of maintaining the vitality of osteocytes. For example, at the time of implant insertion in free fibula or iliac crest bone grafts, most of the biopsies showed partial or total necrotic bone.58 There is also limited atrophy of free fibular grafts after mandibular reconstruction.57,110 The question is raised: How much vital bone is necessary for the survival of the graft?
1.5 Osteoconductive characteristics of autografts
According to the textbooks, autologous bone has the following properties: “osteoconductive, osteogenic, and osteoinductive.”3 Osteoconductive is the term used to describe the property of new bone being able to form on the surface.3 Therefore, osteoconductive materials can not only serve as a guide rail for bone regeneration in a defect of critical size, but also in bone augmentation. It is also a term, albeit unusual, for the property of implant surfaces that allows for the deposition of new bone without the formation of a fibrous layer.3,34 Osteoconductivity therefore first requires a surface. Once the transplanted bone has been partially resorbed, the remaining bone surface again becomes osteoconductive.100 Transplanted bone that remains after the initial resorption phase serves as a guide splint. Clinically, it is therefore common to dimension autologous bone, taking into account partial resorption. The reason why autologous bone clearly allows more bone formation than bone substitutes in the first weeks after transplantation in a pig mandibular defect16 remains a matter of speculation, but it is not particularly surprising that osteoblasts and their mesenchymal progenitors like the mineralized surface that they have produced themselves. In summary, the property osteoconductive can be confirmed for autologous bone through histology.
1.6 Osteogenic properties of autografts
Autografts contain viable osteoprogenitor cells, in contrast to allografts and bone substitutes of xenogeneic and synthetic origin. By definition, osteogenic means that the cells brought along during transplantation actively participate in bone formation, i.e. osteogenic precursor cells of the mesenchymal line differentiate into osteoblasts after transplantation and form new bone. Numerous in vitro studies have shown that osteogenic cells can be generated from explant cultures of bone grafts, particularly from trabecular but also from cortical bone.56,115 The key experiment that proved osteogenicity related to transplantation at ectopic sites. This research took place in the 1970s, when Gray and Elves transplanted isografts from the ilium52 and femur diaphysis,53 e.g. into the back of rats. They showed bone formation after 2 weeks, mainly originating from the transplanted endosteal and periosteal cells. Once the cells were removed by enzymatic digestion or through boiling, the osteogenic capacity was nil, suggesting that the osteocytes could not replace the cells on the surface and that the bone matrix alone could not induce bone formation.
In a xenogeneic transplantation model, 5 mm3 of human morselized cancellous bone from the proximal femur was transplanted into immunodeficient mice that received radiation, and depletion of macrophage and natural killer cells. Consistently, after 8 weeks, new bone was produced by human bone cells rather than from the induction of host mesenchymal cells into mouse osteoblasts.13 Bone transplants, however, underwent resorption and necrosis in untreated immunodeficient mice, considering that macrophages could developed into osteoclasts.13 Also, in a goat model, ectopic transplantation of 1 cm3 of femur condylar corticocancellous bone was transplanted in the paraspinal muscle. Both the block grafts and the respective bone chips showed ectopic new bone formation after 12 weeks. Upon freeze thawing, block grafts maintained a weak osteogenic potential, while the respective bone chips were resorbed,75 probably because only a few osteogenic cells can survive under such conditions.114 Preclinical research in goats showed that it requires a well-nourished environment for the transplanted osteogenic cells to contribute to bone formation.74 Mouse models further revealed that perivascular cells located within transcortical channels contributed to osteoblast formation and bone tube closure in a cortical bone transplantation model.97,123
Recent evidence further suggested that, at least in a mouse model, the interval between autograft harvesting and transplantation affected its viability and bone-forming capacity.119 Immediately after autograft harvesting, apoptotic cells were barely detectable, but already within 5 minutes the number of apoptotic cells had nearly tripled.119 The time between harvesting and transplantation also affected the osteogenic potential of an autograft upon transplantation.119 Overall, autografts resemble the osteogenic potential of tissue engineering constructs, showing ectopic bone formation; however, when in small-sized constructs, only around 20 to 70 mm3 in mice79 and in goats.76 Importantly, however, the orthotopic transplantation could not reveal any benefit of cell-based therapies with respect to bone formation at the defect site.76 Thus, beside the fact that osteogenic cells can basically survive transplantation and are a source of osteoblasts at ectopic sites, the overall contribution of the transplanted cells to graft consolidation is yet to be investigated. Nevertheless, our biopsies suggest that the new bone originates from the transplanted bone chips, with new, nascent bone bridging the space between the transplanted bone particles (Fig 1-12).
1.7 Osteoinductive properties of autografts
The postulated osteoinductive effect attributed to autografts is questionable. By definition, the unlimited osteoinductive effect of autografts can only be proven by ectopic bone formation outside the skeletal system, and not only in or on the bone. Osteoinductive refers to demineralized bone but also dentin matrix, both of which can trigger new bone formation after implantation, e.g. in the muscle of a rat.63 This demineralized bone matrix ultimately leads to the isolation and molecular characterization of bone morphogenetic proteins (BMPs). It is not widely known that the isolation of BMPs requires 5 to 20 kg of bone to obtain sufficient protein for purification testing, including in vitro osteogenic differentiation and in vivo osteoinductive bone formation.8,83,133 However, there is no evidence for ectopic bone regeneration after transplantation of bone chips in a muscle; in fact, it is the opposite – that the bone chips are resorbed without the induction of new bone by host-derived induced osteoblasts.13
Fig 1-12 New bone formation on the surface of transplanted bone chips. In the SBB technique, after 3 months, healing areas of nascent bone formation are detectable. The structure of future bone formation can already be anticipated. Even though the origin of the bone is not defined, it seems that bone formation originates from the transplanted osteogenic cells.
When implanted into the muscle pouches of beagle dogs, bone grafts resorbed quickly, while alloplasts and a synthetic biphasic calcium phosphate showed minor signs of ectopic bone formation.87 Also, in Wistar rats, autologous bone chips from a corticocancellous bone block grafted in a muscle were entirely resorbed after 6 weeks.88 If native bone chips were actually unlimitedly osteoinductive, it would mean that, were bone chips to enter the soft tissue, new bone would be formed there. This side effect of ectopic bone formation in soft tissue would be clinically undesirable. However, there is evidence that, at least during bone remodeling, osteoclasts release TGF-βb1 from the bone matrix, which recruits mesenchymal progenitors to the remodeling sites.32 It was recently confirmed that TGF-β1 released by acid lysis of bone is a major regulator of gene expression in mesenchymal cells in vitro.117 Moreover, acid bone lysates delayed bone formation in a rat calvaria defect model.116 Since the accumulation of these results makes a major involvement of BMPs in graft consolidation unlikely, and proposes that TGF-β1 supports the immigration of progenitor cells, the idea of the osteoinductive properties of autografts needs to revisited, and has to be limited only to the support of bone formation in direct contact with bone tissue.
1.8 Summary
In summary, the surgical approaches outlined in Chapter 4 of this book – the bony lid technique, the SBB technique, and the bone core technique67,68 – nicely reflect the basic principles of successful bone healing introduced in this chapter, which are that:
1. Autografts have favorable osteoconductive properties, allowing bone to form on the surface.
2. Once harvested and used immediately, autografts can exert osteogenic properties where the transplanted cells might contribute to bone formation.
3. The osteoinductive properties of autografts are questionable, and it is desirable to avoid ectopic bone formation at soft tissue sites.
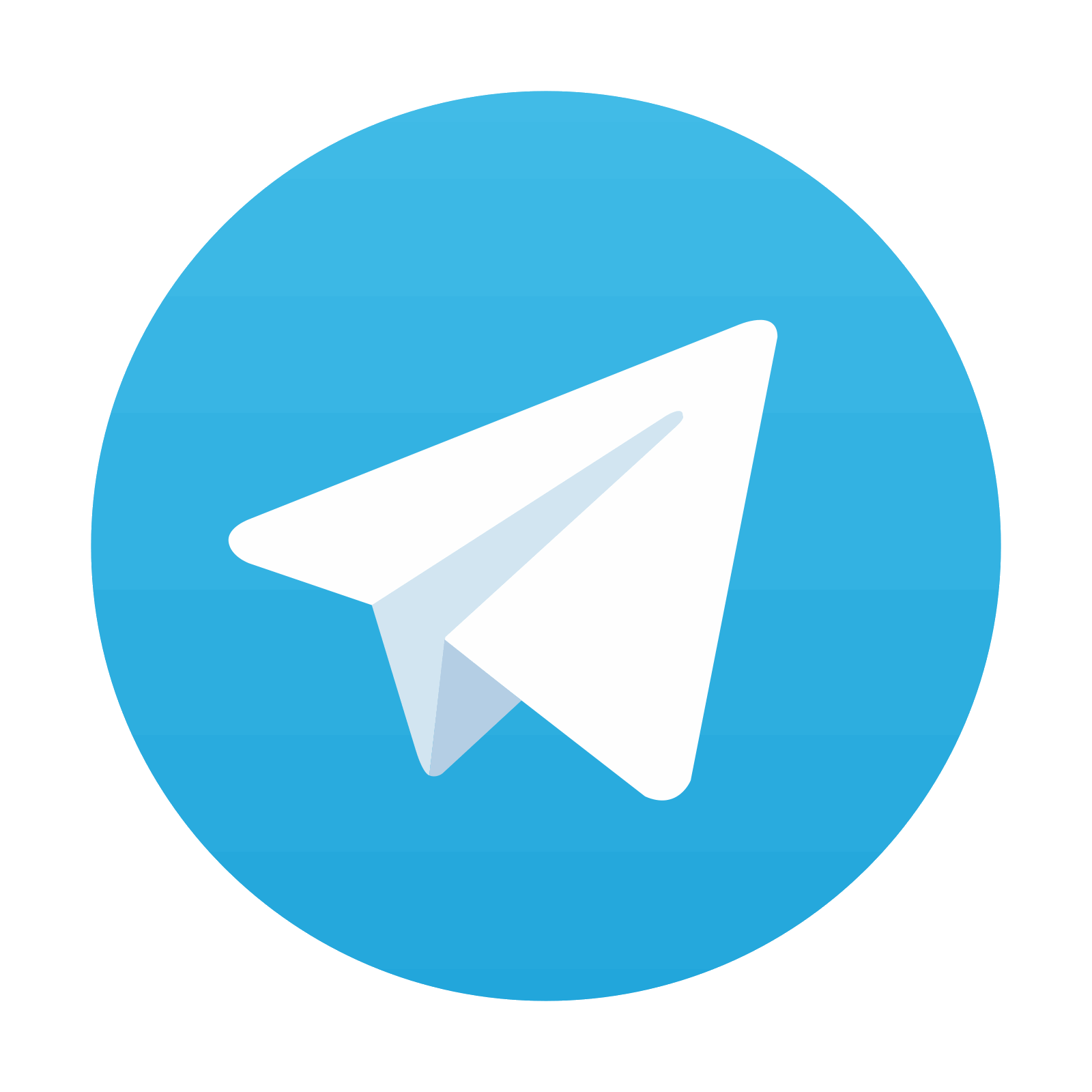
Stay updated, free dental videos. Join our Telegram channel

VIDEdental - Online dental courses
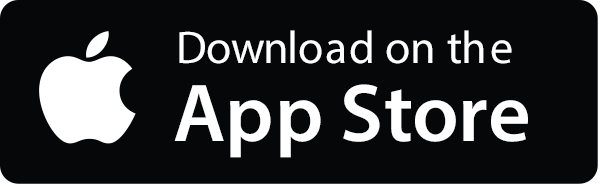
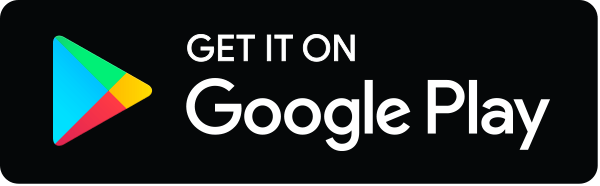