Bioenergetics
Publisher Summary
This chapter discusses the properties of enzymes as catalysts for chemical reactions. ATP occurs in all cells and is a universal intermediate in cell energy metabolism. The base adenine is joined to C-1’ of ribose, the 5’ position of which is linked to a phosphate residue to form adenosine monophosphate (AMP). Addition of a second phosphate group to the first via an anhydride bond gives the compound adenosine diphosphate (ADP), while ATP is derived by the addition of a further phosphate again via an anhydride linkage. As the hydrolysis of the terminal phosphate bond of ATP proceeds with a large negative standard free energy change, ATP is sometimes referred to as a high-energy compound or as possessing high-energy bonds. The function of ATP as an intermediate in energy metabolism depends on the fact that the value of AG for ATP hydrolysis at the concentrations of ATP, ADP, and phosphate present in the cell is large and negative. ATP provides energy for the transport of certain metabolites across cell membranes. In many cells, glucose and neutral amino acids are accumulated across the cell plasma membrane by specific transport systems so that the internal concentration is higher than the external concentration.
The properties of enzymes as catalysts for chemical reactions were considered in Chapter 6. This chapter now considers some of the associated energetic aspects of enzyme reactions in the cell.
Chemical equilibria
< ?xml:namespace prefix = "mml" />

if solutions of A and B are mixed and an appropriate enzyme is added C and D will be formed. Once this happens the back reaction will also take place with the re-conversion of C + D to A + B. Net production of C and D proceeds until the concentrations of substrates and products have adjusted to values where the rate of the back reaction is equal to the rate of the forward reaction. At this point, there will be no further net formation of products and the reaction is said to have attained equilibrium.
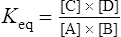
The standard free energy change of a reaction is related to the equilibrium constant as follows:

where R is the gas constant and T is the absolute temperature.

Thus reactions with a high value of Keq’ have a large negative standard free energy change, while reactions with a low Keq’ have a large positive ΔG0’ For reactions with Keq’ near 1, the standard free energy change is near zero. Values of Keq’ together with the corresponding values of ΔG0′ are given in Table 16.1.
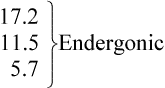
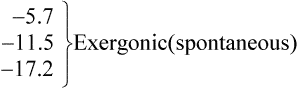

where [A], [B], [C] and [D] are the initial concentrations of reactants and products in the system. If the reactants and products are initially present at their equilibrium concentrations then the above equation reduces to ΔG = 0 and no energy can be obtained from the system.
ATP (adenosine triphosphate)
phosphate group to the first via an anhydride bond gives the compound adenosine diphosphate (ADP) while ATP is derived by the addition of a further phosphate again via an anhydride linkage. At pH 7·4 ATP bears four negative charges and forms a stable complex with Mg2+ ions.
ATP can be hydrolysed enzymically to ADP and inorganic phosphate:

The standard free energy change (ΔG0’) of this reaction is approximately −30 kJ mol−1 and this is high relative to many other hydrolysis reactions (Table 16.2). The high standard free energy of hydrolysis of ATP arises in part from the fact that the products of the reaction, ADP and phosphate, both carry negative charges and hence tend to repel each other. Hydrolysis of the terminal phosphate–phosphate bond of ATP can be regarded as relieving some of the intramolecular strain caused by the presence of neighbouring negatively charged groups in the molecule.
Table 16.2
The standard free energy change (ΔG0’) of hydrolysis of some phosphate-containing compounds
Compound | ![]() |
Phosphoenolpyruvate | −53·5 |
1,3-Diphosphoglycerate | −49·5 |
Phosphocreatine | −44·0 |
ATP | −29·4 |
Glucose 1-phosphate | −21·0 |
Fructose 6-phosphate | −16·0 |
Glucose 6-phosphate | −13·8 |
3-Phosphoglycerate | −13·0 |
Glycerol 1-phosphate | −9·6 |
ATP can also undergo hydrolysis as follows:

Pyrophosphate + H2O → 2 phosphate ΔG°’ = −30 kJ mol−1
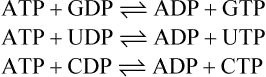
The ATP/ADP cycle
In the cell, ATP can be regarded as undergoing a continuous cycle of breakdown and resynthesis:
A number of other phosphate-containing compounds have standard free energy changes of hydrolysis even greater than that of ATP (Table 16.2). Reactions in which such molecules transfer their terminal phosphate groups to ADP to form ATP are therefore energetically favourable. ATP can be formed in this way from ADP plus phosphoenolpyruvate (page 229), ADP plus creatine phosphate (page 331) and ADP plus 1,3-diphosphoglycerate (page 228). All these are important so-called substrate level phosphorylation reactions although far more ATP is synthesized by oxidative phosphorylation and the respiratory chain.
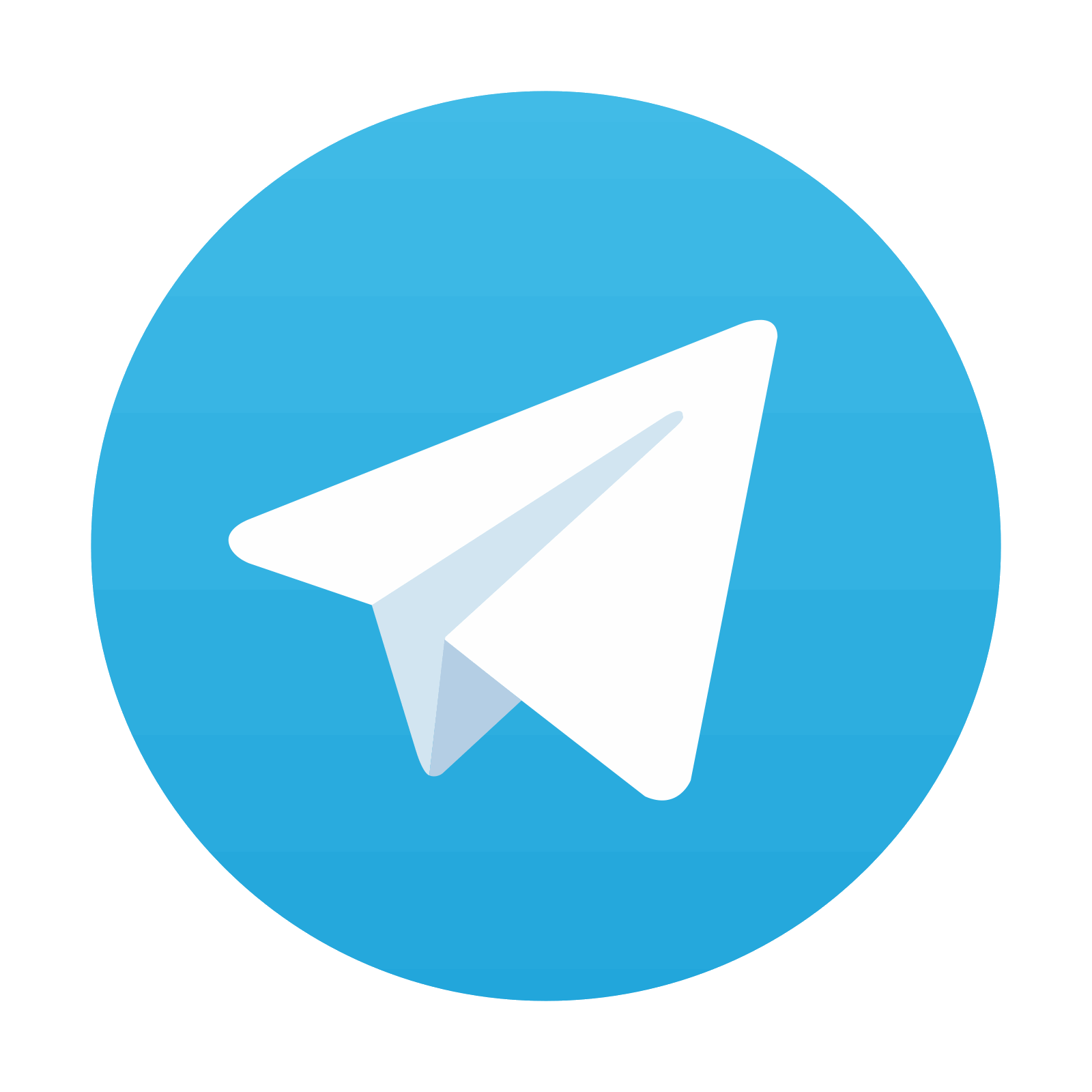
Stay updated, free dental videos. Join our Telegram channel

VIDEdental - Online dental courses
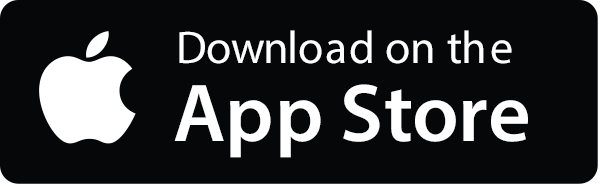
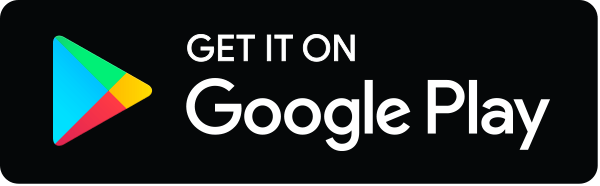