Progressive Bone Loading
Increasing the Density of Bone with a Prosthetic Protocol
Carl E. Misch
The surgical and prosthetic protocols for the development of a predictable direct bone–implant interface with root-form implants were developed and reported by Brånemark et al.1 After an initial direct bone–implant interface has been obtained and confirmed at the posthealing stage II surgery, the implant is most at risk for failure or crestal bone loss within the first year after loading.2–8 Failure and crestal bone loss occurs primarily from excessive occlusal stress or poor bone strength at the interface during early implant loading. If the treatment plan provides adequate support, the three most common causes of early prosthetic-related implant complications are nonpassive superstructures, partially unretained restorations, and loading of the implant support system beyond the strength of the bone–implant interface.9,10
The external (cortical) and internal (trabecular) structure of bone may be described in terms of quality or density, which reflects a number of biomechanical properties, such as strength, modulus of elasticity, bone implant contact percent, and stress distribution around a loaded endosteal implant (Box 32-1). The density of available bone in an edentulous site is a determining factor in treatment planning, surgical approach, implant design, healing time, and the need for initial progressive bone loading during prosthetic reconstruction.11–15
Influence of Bone Density on Implant Success Rates
Several independent groups have reported different failure rates related to the quality of the bone. In fact, the early implant failure most often is more related to bone density than arch location. For example, Zarb and Schmitt reported early loading failures in 3.3% of primarily completely edentulous mandibular patients with good-quality bone.16 Naert and Quirynen observed a 2.5% early loading failure rate in partially edentulous patients.8 Salonen et al. reported a 3.9% failure rate in a study of 204 implants.7
On the other hand, a more early loading failure is also reported, especially in softer bone types. For example, Johns et al. reported 3% failure of implants in moderate bone densities but a 28% implant failure in the poorest bone type.17 Engquist et al. observed that 78% of all reported implant failures were in soft bone types when they supported overdentures.18 Friberg et al. observed that 66% of implant failures occurred in the resorbed maxilla with soft bone.19 Jaffin and Berman, in a 5-year report, observed a 44% implant failure when poor density bone was observed in the maxilla and 35% implant loss in any region of the mouth when bone density was poor, with 55% of all implant failures in the soft bone type.4 Smedberg et al. reported a 36% failure rate in the poorest bone density.20
Hermann et al. found that implant failures were strongly correlated to patient factors, including bone quality, especially when coupled with poor bone volume (65% of these patients experienced failure).21 Schnitman et al. reported 22% failure in the soft bone of the posterior maxilla and a 0% failure in the good bone of the anterior mandible during a 3-year period3 (Figure 32-1). Jemt et al., reported early implant failures as great as 35%, especially in poor bone quality, after successful surgical survival of implants.5 Sullivan et al. reported late failures in 7% of the maxillae and 1.4% of mandibles after reverse torque testing had identified 6.4% maxillary and 3.2% mandibular implant failures at stage II recovery.22
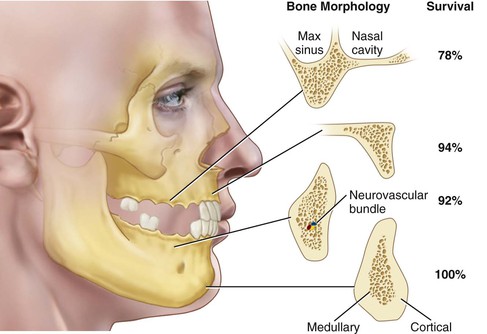
These reported failures are not primarily related to surgical healing but instead occur after prosthetic loading. In other words, early loading failure rate has a wide range of survival, with many reports noting that poor bone density dramatically increases the risk of failure. Therefore, over the years, many independent clinical groups, following a standardized surgical and prosthetic protocol, documented the influence of bone density on clinical success.23–25
In a report of 22,177 implants, Root Laboratory found that 5.9% of the implants were lost from the final impression to the initial delivery of the prosthesis.26 The 22,177 implants were used to restore 7403 patients, and each implant prosthesis averaged three implants. The number of prostheses affected by 5.9% implant failure was 15% of the restorations (Table 32-1). In other words, when early implant failure is reported, the number of restorations affected may be multiplied by approximately three times. Hence, early loading failure is a considerable risk to both patient and restoring dentist.
TABLE 32-1
Implant versus Prostheses Failure
Total Root Forms* | |
7403 patients | 22,177 implants |
7403 patients | 1107 (15.0%) lost one or more* |
22,177 fixtures | 1319 (5.9%) lost* |
* Loss occurred between final impression and time of loading.
Data from Root Laboratory: Statistics report on implant and prosthesis failure during the first year, Leawood, KS, 1992, Root Laboratory.
Misch first proposed the concept of progressive or gradual bone loading during prosthetic reconstruction to decrease early implant failure in 1980 based on empirical information.27 The theory was that bone reacts to mechanical stress similar to a muscle. When it is not stimulated, the muscle (bone) becomes weaker. It was logical to assume when the muscle (bone) is stimulated, the muscle (bone) will become stronger.
A protocol established by the author in 1988, which adapts the treatment plan, implant selection, surgical approach, healing regimen and initial prosthetic loading, has resulted in similar implant success rates in all bone densities and all arch positions.12–15 Misch et al. reported on 364 consecutive implants in 104 patients with 98.9% survival at stage II uncovery followed by a progressive loading format and found no early loading failures during the first year of function.28 In another report by Misch et al., no early loading failures were observed in the posterior maxilla over a 5-year period with 453 implants and 131 prostheses using a bone density–based implant design and progressive bone loading.29 A 10-year report by Misch et al. of short implants using progressive loading observed a 99.5% success rate during this time frame.30 Kline et al. also reported a 99.5% success rate at 5 years for 495 implants following a similar progressive loading approach31 (Table 32-2).
TABLE 32-2
Early Loading Failure in Soft Bone
Reference | Early Loading Failure (%) |
Johns et al.17 | 28 |
Jaffin and Berman4 | 35 |
Smedberg et al.20 | 36 |
Jemt et al.5 | 35 |
Misch et al.27 | 0 |
Misch et al.29 | 0 |
Kline et al.31 | 1 |
Misch et al.30 | 1 |
Over the years, the author has evaluated and modified this concept to incorporate time intervals, diet, occlusion, prosthesis design, and occlusal materials. In addition, it was noted that early crestal bone loss in softer bone types was also reduced with a gradual loading process.32,33 This chapter presents the aspects of bone density related to progressive loading of an implant prosthesis.
Etiology of Variable Bone Density
Bone is an organ that is able to change in relation to a number of factors, including hormones, vitamins, and mechanical influences. However, biomechanical parameters, such as the amount of strain transmitted to bone, are predominant.34 Awareness of this adaptability in the skeletal system has been reported for more than a century. In 1887, Meier qualitatively described the architecture of trabecular bone in the femur.35 In 1888, Kulmann noticed the similarity between the pattern of trabecular bone in the femur and stress trajectories in construction beam concepts used by Eiffel36 (Figure 32-2). Wolff, in 1892, further elaborated on these concepts and published, “Every change in the form and function of bone or of its function alone is followed by certain definite changes in the internal architecture, and equally definite alteration in its external conformation, in accordance with mathematical laws.”37 The modified function of bone and the definite changes in the internal and external formation of the vertebral skeleton as influenced by mechanical load were also reported by Murry.38
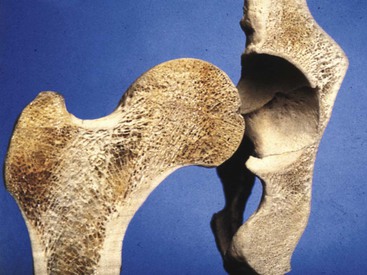
When bone is not stimulated enough, the bone mass and size are reduced. This phenomenon occurs throughout the skeletal system as evidenced by a 15% decrease in the cortical plate and extensive trabecular loss to bone immobilized for 3 months.39 Cortical bone decrease of 40% and trabecular bone decrease of 12% also have been reported in response to disuse.40,41 On the other hand, bone also can respond to increased stress, if within physiologic limits, by an increase in density.42 Dahlin and Olsson report an increase in cortical bone thickness and overall mineral content under stressful stimuli.43 Weight lifters have skeletal systems with larger diameter and more dense bone. Professional tennis players have larger-diameter wrists, humeri, and ulnas on their dominant playing arms. Hence, bone loss or gain may occur in relation to the amount of strain to the skeletal system.
The structural changes in bone as a consequence of mechanical influences have also been noted in the jaws. As example, both MacMillan and Parfitt have reported on the structural characteristics and variation of trabeculae in the alveolar regions of the jaws.43,44 The maxilla and mandible have different biomechanical functions (Figure 32-3). The mandible, as an independent structure, is designed as a force absorption unit. Therefore, when teeth are present, the outer cortical bone is denser and thicker, and the trabecular bone is more coarse (Figure 32-4). On the other hand, the maxilla is a force distribution unit. Stresses to the maxilla are transferred by the zygomatic arch and palate away from the brain and orbit. As a consequence, the maxilla has a thin cortical plate and fine trabecular bone surrounding the teeth (Figure 32-5).
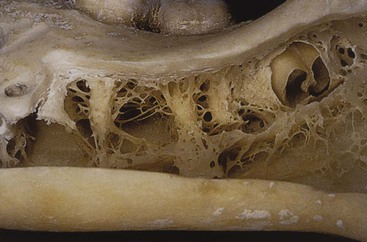
Neufeld noted that the bone is most dense around the teeth (cribriform plate) and more dense around the teeth at the crest compared with the regions around the apices45 (Figure 32-6). Alveolar bone resorption associated with orthodontic therapy also illustrates the biomechanical sensitivity of the alveolar processes.46,47 Orban demonstrated a decrease in the trabecular bone pattern around a maxillary molar with no opposing occlusion compared with a tooth with occlusal contacts on the contralateral side48 (Figure 32-7).
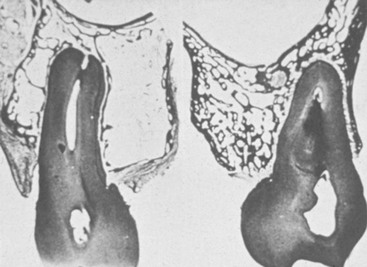
Bone density in the jaws also decreases after tooth loss. This loss is primarily related to the length of time the region has been edentulous and not appropriately loaded, the initial density of the bone, flexure and torsion in the mandible, and parafunction before and after tooth loss.49–51 In general, the density change after tooth loss is greatest in the posterior maxilla and least in the anterior mandible.
Misch Bone Density Classification
Dense or porous cortical bone is found on the outer surfaces of bone and includes the crest of an edentulous ridge. Coarse and fine trabecular bone types are found within the outer shell of cortical bone and occasionally on the crestal surface of an edentulous residual ridge. These four macroscopic structures of bone may be arranged from the most dense to the least dense, as first described by Frost and Roberts: dense cortical bone, porous cortical bone, coarse trabecular bone, and fine trabecular bone34,52 (Figure 32-8). In combination, these four macroscopic densities constitute the four bone categories described by Misch (D1, D2, D3, and D4) located in the edentulous areas of the maxilla and mandible13 (Figure 32-9). The regional locations of the different densities of cortical bone are more consistent than the highly variable trabecular bone.
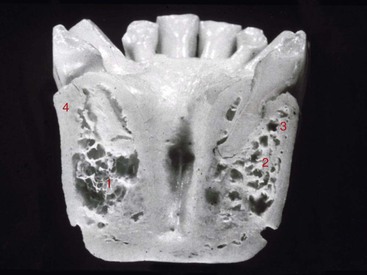
D1 bone is primarily dense cortical bone. D2 bone has dense-to-porous cortical bone on the crest and lateral to the implant site. The bone within this cortical housing has coarse trabecular bone (Figure 32-10). D3 bone types have a thinner porous cortical crest and facial and lingual regions, with fine trabecular bone in the region next to the implant (Figure 32-11). D4 bone has almost no crestal cortical bone and porous cortical lateral plates. The fine trabecular bone comprises almost all of the total volume of bone next to the implant (Figure 32-12). The bone density may be determined by the general location, tactile sense during surgery, or computerized radiographic evaluation.
Scientific Rationale of Bone Density–Based Implant Treatment
Numerous investigators have generated in vitro data to determine the direct relationships of elastic modulus, structural density, and ultimate strength of bone to the relative density of bone.
Bone Strength and Density
Bone density is directly related to the strength of bone before microfracture.53 The bone densities that originally relied on clinical impression are now fully correlated to quantitative objective values obtained from computed tomography scans and bone strength measurements. These values can help prevent failure in specific situations of weak densities. Misch et al. reported on the mechanical properties of trabecular bone using the Misch density classification.54 A 10-fold difference in bone strength may be observed from D1 to D4 bone (Figure 32-13). D2 bone exhibited a 47% to 68% greater ultimate compressive strength compared with D3 bone. In other words, on a scale of 1 to 10, D1 bone is a 9 to 10 relative to strength, D2 bone is a 7 to 8 on this scale, D3 bone is 50% weaker than D2 bone and is a 3 or 4 on the strength scale and D4 bone is a 1 to 2 and up to 10 times weaker than D1 bone (Box 32-2).
It should be noted that the strength of bone studies were performed on mature bone types. Bone is 60% mineralized at 4 months after implant surgery, and the strength of bone is related to the amount of mineralization. Hence, it is rational to wait longer before loading an implant when the bone density is D3 or D4. A healing period after surgery of 3 to 4 months is adequate for D1 and D2 bone. A healing period of 5 to 6 months is beneficial in D3 to D4 bone.
Elastic Modulus and Density
The elastic modulus describes the amount of strain (changes in length divided by the original length) as a result of a particular amount of stress. It is directly related to the apparent density of bone.55 The elastic modulus of a material is a value that relates to the stiffness of the material. The elastic modulus of bone is more flexible than titanium. When higher stresses are applied to an implant prosthesis, the titanium has lower strain (change in shape) compared with the bone. The difference between the two materials may create microstrain conditions of pathologic overload and cause implant failure. When the stresses applied to the implant are low, the microstrain difference between titanium and bone is minimized and remains in the adapted window zone, maintaining load-bearing lamellar bone at the interface.56
Misch et al. found the elastic modulus of the trabecular bone in the human jaw to be different for each bone density54 (Figure 32-14). As a result, when a stress is applied to an implant prosthesis in D1 bone, the titanium–D1 bone interface exhibits very small microstrain difference. In comparison, when the same amount of stress is applied to an implant in D4 bone, the microstrain difference between titanium and D4 bone is greater and may be in the pathologic overload zone (Figure 32-15). As a result, D4 bone is more likely to cause implant mobility and failure. Conclusions agree with prior reports and show the importance of bone quality in the treatment planning and early loading phase for improved long-term prognosis.
Bone Density and Bone–Implant Contact Percentage
The initial bone density not only provides mechanical immobilization of the implant during healing but after healing also permits distribution and transmission of stresses from the prosthesis to the implant–bone interface. The mechanical distribution of stress occurs primarily where bone is in contact with the implant. Open marrow spaces or zones of unorganized fibrous tissue do not permit controlled force dissipation or microstrain conditions to the local bone cells. Because stress equals force divided by the area over which the force is applied, the less the area of bone contacting the implant body, the greater the overall stress, other factors being equal. Therefore, the bone–implant contact (BIC) percent may influence the amount of stress and strain at the interface.
In 1990, Misch noted that the bone density influences the amount of bone in contact with the implant surface, not only at first-stage surgery but also at the second-stage uncovery and early prosthetic loading.13 The BIC percentage is significantly greater in cortical bone than in trabecular bone. The very dense D1 bone provides the highest percentage of bone in contact with an endosteal implant and may approximate more than 85% BIC (Figure 32-16). D2 bone, after initial healing, usually has 65% to 75% BIC. D3 bone typically has 40% to 50% BIC after initial healing (Figure 32-17). The sparse trabeculae of the bone often found in the posterior maxilla (D4) offer fewer areas of contact with the body of the implant. With a machined-surface implant, this may approximate less than 30% BIC and is most related to the implant design and surface condition (Figure 32-18).
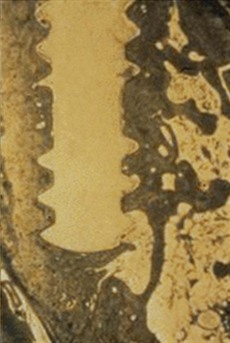
Consequently, greater implant surface area is required to obtain a similar amount of BIC in soft bone compared with a denser bone quality.6 As a result, many anterior mandibles with more dense bone have less importance of implant number, size, implant design, or progressive loading compared with posterior maxillae with less dense bone.
Bone Density and Stress Transfer
Crestal bone loss after loading may occur from excess stress at the implant–bone interface.32,33,57 A range of marginal bone loss has been observed in implants in different bone densities with similar load conditions. In the study by Manz, the loss of central bone around an implant loaded for 6 months after stage II uncovery was directly related to the density of the bone. D1 bone lost the least marginal bone and D4 bone lost the most during the 6-month period.58 Misch and Bidez noted in 1990 that part of this phenomenon may be explained by the evaluation of finite element analysis (FEA) stress contours in the different volumes of bone for each bone density.59
Early implant failure may also be related to the difference in stress transfer between different bone densities. Misch and Bidez performed a three-dimensional finite stress element analysis on patients with bone volumes of division A, B, and C minus width.59 Each volume of bone consisted of cortical and trabecular models with four macroscopic bone densities corresponding to D1 (100%), D2 (75%), D3 (50%), and D4 (25%). The authors observed a correlation between bone volume strength and density. Clinical failure was mathematically predictable in all D4 bone models and two of the D3 densities, depending on the bone volume.
Other studies using FEA models with various implant designs and bone quality have also evaluated the stress–strain distribution in the bone around the implants.60,61 For example, Tada et al. evaluated the three-dimensional changes around different length implants in different bone qualities62 (Figure 32-19). The type 3 and 4 bone categories had four to six times more strain around all implants, with the highest strains around the shortest implants.
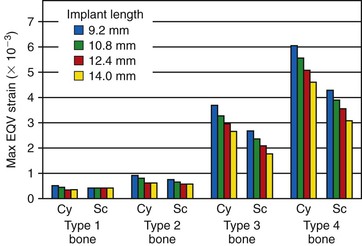
As a result of the correlation of bone density to the elastic modulus and BIC percent, when a load is placed on an implant, the stress contours in the bone are different for each bone density.63 In D1 bone, the highest strains are concentrated around the implant near the crest, and the stress in the region is of lesser magnitude. D2 bone, with the same load, sustains a slightly greater crestal strain, and the intensity of the stress extends farther apically along the implant body. D4 bone exhibits the greatest crestal strains, and the magnitude of the stress on the implant proceeds farthest apically along the implant body.
Hence, dental literature review of failures, orthopedic literature of bone remodeling, literature regarding bone mechanics of the jaws, and FEA suggest the softer bone types have higher risk of implant failure.64 As a consequence of different elastic modulus, BIC, strain regions found around implants with different bone densities, and strength of bone related to density, the magnitude of a prosthetic load may remain similar and yet give one of the following three different clinical situations at the bone–implant interface based on the bone density around the implant: (1) physiologic bone loads in the adapted window zone and no marginal bone loss, (2) mild overload to pathologic overload bone loads and crestal bone loss, or (3) generalized pathologic overload and implant failure. Therefore, to obtain a similar clinical result in each implant prosthesis, the variables in each patient must be either eliminated, reduced, or accounted for in the treatment plan.
Because the myriad of variables cannot be eliminated relative to bone density, the treatment plans (including implant number, size, and design) should be modified. Progressive bone loading for the softest bone types also increases bone density and its associated mechanical factors of strength, modulus of elasticity, BIC, and stress transfer.
Rationale for Progressive Loading
Bone Physiology
Cortical and trabecular bone throughout the body are constantly modified by either modeling or remodeling.65 Modeling has independent sites of formation and resorption and results in the change of the shape or size of bone. Remodeling is a process of resorption and formation at the same site that replaces previously existing bone and primarily affects the internal turnover of bone, including the region where teeth are lost or the bone next to an endosteal implant. These adaptive phenomena have been associated with the alteration of the mechanical stress and strain environment within the host bone.66
Bone responds to hormonal and biomechanical regulation.67 However, even in instances in which the demand for calcium is great (the primary objective for hormonal regulation), functional loading can compete and maintain bone mass.68 Most women are aware of increased risk of osteoporosis after menopause and hormonal changes, yet bone loss (or gain) is more controlled by mechanical factors than hormones. Even in an animal with calcium levels in the blood so low that death may occur, when the limbs are exercised, the bone takes the calcium from the blood to increase its strength, and the animal dies. In other words, the mechanical stimulation of bone can cause cellular events that can even override life.69
Stress is determined by the magnitude of force divided by the functional area over which it is applied. Strain is defined as the change in length of a material divided by the original length. The greater the magnitude of stress applied to the bone, the greater the strain observed in the bone.70 Bone modeling and remodeling are primarily controlled, in part or whole, by the mechanical environment of strain. Overall, the density of trabecular bone evolves as a result of mechanical deformation from microstrain. The deformation of the alveolar bone by mechanical forces even is related to the thickness of the bony plate.
Clinical evaluation confirms an increase in the amount of trabecular bone and cortical plate thickness in patients with natural teeth exhibiting parafunction. A dentist can observe these bone density changes when attempting to extract teeth. In a severely parafunctional patient, the tooth usually fractures when attempting to remove it because the surrounding bone is stronger than the tooth. In a maxillary posterior second molar unopposed by any mandibular tooth, the bone is so soft that the complete tuberosity and surrounding bone fractures and the tooth and attached surrounding bone are removed as one piece.
Frost proposed that bone mass is a direct result of the mechanical usage of the skeleton.52,71–73 He redeveloped a mechanical adaptation chart relating trivial loading, physiologic loading, overloading, and pathologic loading zones to ranges of microstrain. His studies demonstrated increases in cortical bone mass related to strains applied to the bone (Figure 32-20). These categories also may be used to describe the trabecular bone response next to a dental implant in the jaws.56,74,75
The actual strain perceived by the bone tissue initiates a chain reaction of events that result in a biological response. Cowin and Hegedus proposed potential mechanisms by which bone cells sense mechanical load.76,77 They suggested that cell-level strains were almost 10 times greater than tissue-level strains. The proposed cellular mechanisms include membrane deformation, intracellular action, and extracellular action.
Bone cells and the extracellular matrix comprise the strain-sensitive population, and each plays a vital role in the mediation of the interface. A review of the literature of in vivo and in vitro studies has shown that dynamic or cyclic loading is necessary to cause a significant metabolic change in the bone cell population.78–84 The greater the rate of change of applied strain in bone, the more bone formation is increased.85 The effect of applied strains on bone is dictated not only by the rate of the applied load but also by the magnitude and duration.
In other words, the prosthetic loading of the dental implant changes the number and density of bone cells. Cyclic loading is necessary to cause a significant metabolic change in bone cell population. Lower magnitude loads applied for many cycles can cause the same anabolic effects of larger loads applied for a limited number of cycles.80 Therefore, a range of clinical conditions may equate to an increase in bone density, with prosthetic loading a logical condition for a dental implant.
Clinical Studies Supporting Progressive Loading
The biomechanical environment plays an intricate role in the quality and compositional outcome of the new implant-to-bone interface. Under loading, bone behaves as a structure with material and architectural properties and as a biological system.86 Functional loading of the implant brings additional biomechanical influences, which greatly affect its maturation. Computer-aided assessment of fixated implants through digital subtraction radiographic image analysis and an interactive image-analysis system demonstrate an increase in density of periimplant bone structures over a 6-month to a 4-year period after the implant was placed.87,88 The major changes of bone condensation around the implants occurred after the first 2 years they were loaded (Figure 32-21). Bone density increase is primarily reflective of the local stress factors, and endosteal implants are the major method to alter the strain and increase bone density in the edentulous jaws.89 Continuously loaded implants remain stable within the bone with bone formation in areas under compression and the orientation of trabeculae corresponding to lines of stress.89
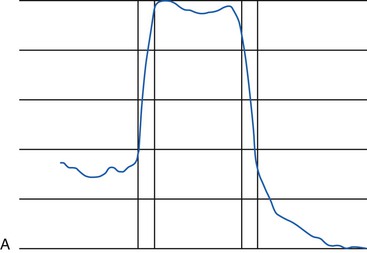
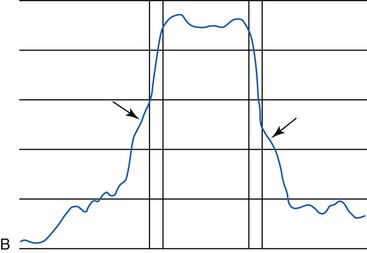
As bone responds to physiologic forces, a gradual increase in loads during prosthetic fabrication stimulates an increase in density. Pierazzini et al. has demonstrated the development of denser trabeculae around progressively loaded implants in animals.90 Piattelli et al. performed histologic and histomorphometric study of bone reaction to unloaded and loaded nonsubmerged single implants in monkeys.91 The bone interfaces of six implants, three loaded and three nonloaded, were evaluated after 15 months. Thicker regions of lamellar cortical bone appeared around the loaded implants compared with unloaded implants (Figure 32-22). The major bone increase in density and amount was observed in the crestal region around the loaded implants. Orthopedic implants causing a change in the loading environment elicited trabecular growth and realignment within the marrow space.92,93
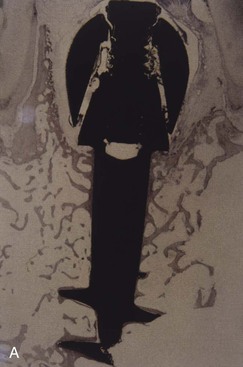
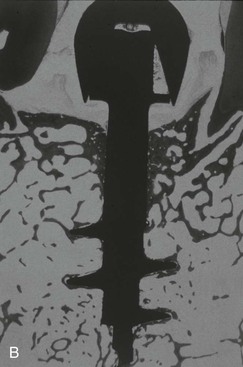
The author evaluated clinical assessment of progressive loading guidelines for 250 implants over a 2-year period using a Periotest.94 This instrument evaluates the dampening effect of implants, prostheses, and teeth, which is related directly to mobility. The Periotest values can range from −8 to +99. A range o/>
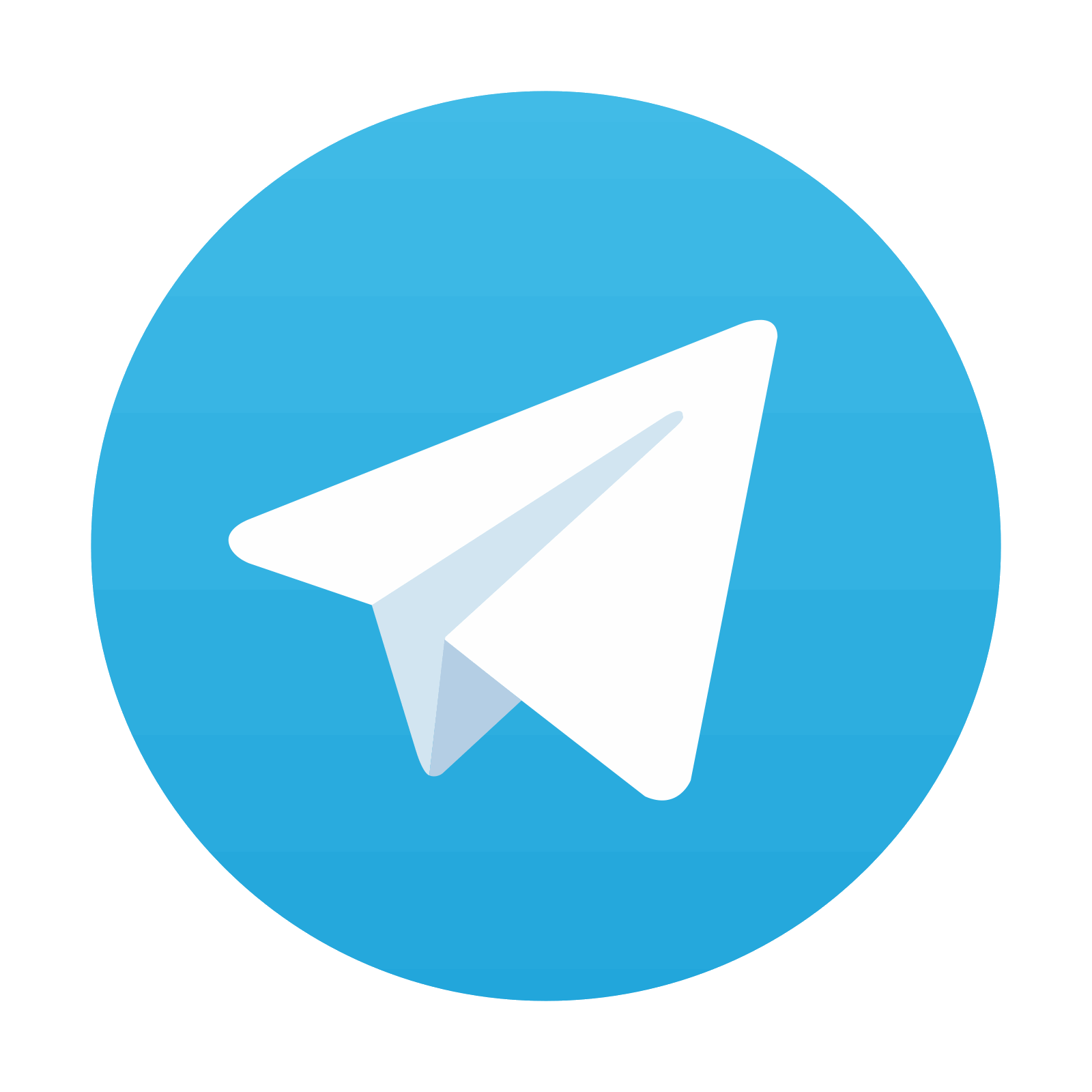
Stay updated, free dental videos. Join our Telegram channel

VIDEdental - Online dental courses
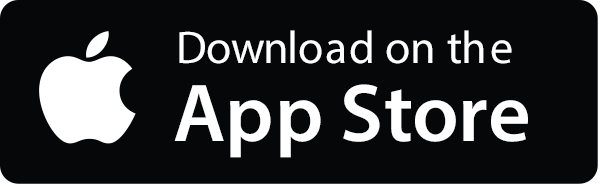
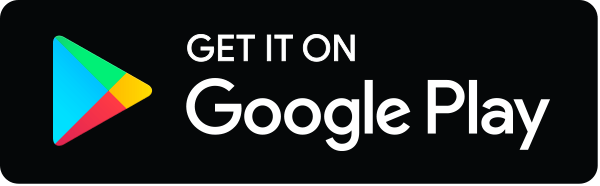