17 Laser Dentistry Research
Optical Coherence Tomography
The history of dental imaging began in the late 1800s with the development of the x-ray image. In 1973, computed tomography (CT) created images by combining x-ray and computer technology to capture thin slices of tissue.1 After that, magnetic resonance imaging (MRI) allowed soft tissue analysis.
Periapical and cephalometric radiographs have been the most important instruments in dental radiology to detect primary and secondary caries, analyze specific anatomical characteristics and structures, plan surgical procedures in implantology, diagnose possible alterations in the bone, and supervise patient progress. However, disadvantages include the interposition of anatomical parts as well as the potential detrimental effects on biological tissues produced by the ionizing radiation.2
Recent developments in the field of optical engineering offer new optical techniques for biomedical imaging applications.2 In addition to the increased availability of compact, modular diode light sources, highly sensitive detectors make it possible to distinguish very small numbers of photons after they interact with the tissue.
The term tomography was first used to describe a sectional radiographic technique in which the x-ray tube moved along in the same plane as the film, but in opposite direction. The image of a selected anatomical plane remains stationary on the moving film while the shadows of all other planes are blurred out of view. The resultant tomographic image is a slice or cross section of the structure. Tomographic images created by CT and panoramic radiography result from the interaction of biological tissues with x-ray photons and represent a selected “layer” or “slice” of the structure using the images recorded.2
Optical coherence tomography (OCT) is a well-established diagnostic imaging technique that has many potential dental applications. OCT is safe, versatile, inexpensive, noninvasive, and readily adapted to the dental office (Figure 17-1). Based on the principles of interferometry, OCT utilizes light from the nonionizing part of the electromagnetic spectrum along with biomedical optics to provide cross-sectional images of tissue up to 3 mm in depth. OCT displays microstructural details that cannot be obtained with current imaging modalities1,3 (Figure 17-2). This concept of using light and optics to image biological tissues was first proposed by Duguay in 1971. After its first biological application, by Huang et al.4 in 1991, OCT was initially applied to tomographic imaging of transparent tissue in the eye for diagnosing retinal macular disease.5–7
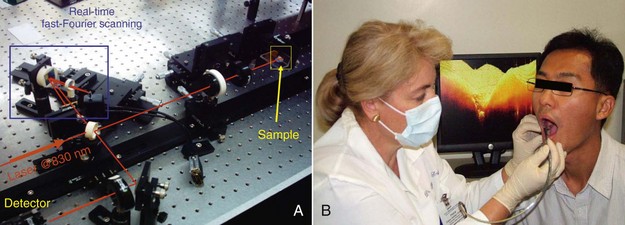
FIGURE 17-1 • A, Real-time optical coherence tomography (OCT) imaging prototype. B, Real-time OCT imaging with the handpiece probe.
(A, Courtesy Professor Anderson Zanardi de Freitas; B, courtesy Dr. Petra Wilder-Smith.)
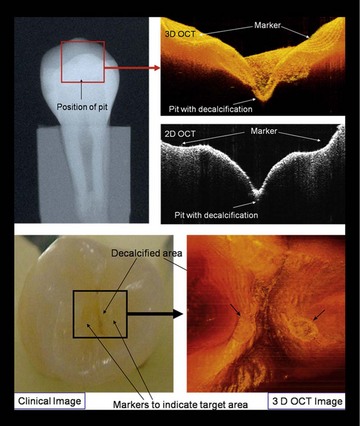
FIGURE 17-2 • Two-dimensional (2D) and three-dimensional (3D) OCT images with clinical intraoral and x-ray comparisons.
(Courtesy Dr. Petra Wilder-Smith.)
Otis et al.2 presented the first intraoral OTC prototype for dentistry in 2000. The system created cross-sectional images by quantifying the reflections of infrared (IR) light from dental structures interferometrically. It consisted of a computer, compact diode light source, photodetector with associated electronics, and handpiece that scanned a fiberoptic cable over the oral tissues. In OCT images the structures appear without the superimposition of other anatomical structures. The final OCT image consists of many axial signal arrays presenting a two-dimensional representation of the tissue reflections. Images can be viewed in real time and stored digitally.2,3
Although OCT is not yet widely clinically available in dentistry, the technique promises fast technological development.8 Applying OCT to other clinically relevant biological structures has been complicated because of optical scattering problems. The oral cavity presents relevant biological tissues close to the surface, so OCT is a promising technique for obtaining images of human dental tissue in vivo.
Despite the significant gains in reducing its incidence, dental caries remains the principal cause of tooth loss worldwide. New treatment approaches emphasize early detection followed by minimal intervention. The most common resource for dental caries diagnosis is the dental radiograph in conjunction with visual and tactile exploration.7 However, these routine procedures are not presently precise enough to diagnose early lesions or gaps at the tooth-restoration interface, which lead to secondary caries.8 The early detection of restoration failures in the tooth-restoration interface could be the first step in preventing secondary caries formation and development, as well as hypersensitivity of restored teeth, development of pulpal pathology, marginal staining, and the ultimate breakdown of a restoration.
Also, intraoral radiographs are highly sensitive and specific to diagnose primary caries but are less reliable in the detection of recurrent caries around existing restorations.2 OCT offers a potentially more sensitive method for detecting recurrent caries. In 2005, de Araujo et al.8 showed the potential of OCT in clinical diagnosis compared with x-ray films. The 10-micron longitudinal resolution of the OCT system identified 50 µ of induced gaps. The failure gaps were not shown by conventional radiographs. According to the authors, OCT has the advantage of showing the restored region as well as the gap, precisely localizing its position.
Amaechi et al.9 quantitatively assessed the mineral changes in a carious lesion based on the effectiveness of preventive measures to remineralize the lesion at an incipient stage. They monitored the changes over time in the mineral status of the caries by using OCT with a system that could collect A-scans (depth vs. reflectivity curve), B-scans (longitudinal images), and C-scans (transverse images at constant depth). Bovine teeth were subjected to demineralization in acidic buffer solution for 3 days, with images taken before demineralization and after 3 days of demineralization. Whereas the B- and C-scans qualitatively described the lesion, the A-scans showed the depth (mm)–resolved reflectivity (dB) of the tooth tissue and were used for the quantitative analysis. The results showed that R (dB mm) decreased with increasing demineralization time, and that the percentage reflectivity loss (R%) in demineralized tissue (amount of mineral loss) increased with increasing demineralization time, showing that OCT could quantitatively monitor the mineral changes in a carious lesion over the long term.
Prosthetic materials (e.g., metal, composites, ceramic fillings/crowns) have also been imaged with OCT, showing its potential advantage over conventional methods by visualizing structural and marginal restoration defects before significant leakage occurred, thus minimizing tooth loss and decreasing replacement restorations.2,7 Although accessibility of the probe tip to the area of interest is likely the limiting factor in this application, OCT also easily identifies marginal adaptation of the metal coping to the cavosurface margin and visualizes the internal aspects and marginal adaptation of porcelain and composite restorations.
In periodontics, because of the microstructural detail of the periodontal soft tissues, OCT offers the potential for identifying active periodontal disease before significant alveolar bone loss occurs. Visual recordings of periodontal tissue contour, sulcular depth, and connective tissue attachment are possible. OCT is a powerful method for generating high-resolution, cross-sectional images of oral structures, with in vivo imaging studies showing much more structural detail of dental tissues compared with previous measurements.2,7
In endodontics the OCT probe can be used to obtain a detailed microscopic image through the surrounding root canal circumferential wall to the outside cementum layer of the root.10 The probe can also image the anatomy and cleanliness of the canal walls and measure the exact thickness of the dentinal wall, which helps prevent canal overpreparation and perforation of the canal walls. OCT could also prove extremely useful in the diagnosis of vertical fractures.11
Alexandrite Laser
The alexandrite laser is a solid-state laser employing a gemstone called alexandrite.12 The alexandrite laser (chrysoberyl [BeAl2O4] doped with chromium ions [Cr3+]) has been a commercially available laser system for medical applications13 and can be pumped with pulsed flashlamps, continuous arc lamps, or laser diodes.14 Its primary wavelength is 752 nm. A tuning range from 710 to 820 nm is possible under optimum operating conditions. A frequency-doubled alexandrite laser (2ω) is possible using a converting medium. A barium beta-borate (β-BaB2O; BBO) crystal is used to create frequency doubling from 752 to 376 nm. At this wavelength, there is good absorption in water and hydroxyapatite, which may be exploited for preparation of dental hard tissue15–19 (Figures 17-3 and 17-4).
In medicine, alexandrite lasers have been used especially for dermatological procedures, such as professional and traumatic tattoo removal, nevus of Ota removal, leg vein treatment, and hair removal.20–23 For hair removal, its clinical use is well established because of its suitable wavelength, which is in the midrange of the melanin-absorbing spectrum and targets the melanin of the hair.21
In dentistry, use of the alexandrite laser has been evaluated in different specialties (e.g., periodontics, endodontics) for amalgam tattoo removal and also for hard tissue removal. In 1991 and 1992, Rechmann, Hennig, and co-workers18,24 first demonstrated that a fast and effective ablation of carious tooth structure was possible using a frequency-doubled Alexandrite laser (377 nm, 100-200–nsec pulse duration, pulse repetition rate up to 110 Hz). In 1994, Jennett et al.25 studied the use of the alexandrite laser (750 nm; 200 µsec/pulse, 700 mJ/pulse, 350 J/cm2 at the surface, 0.25 Hz). Knoop hardness and scanning electron microscopy (SEM) studies were performed on the treated samples. The dye of choice was indocyanine green (ICG) because its absorption peaks lies directly in the alexandrite wavelength spectrum, with three concentrations tested (0.5%, 1.0%, 1.5%). The authors observed no signs of ablation when dye was not used with the wavelength of 750 nm. The deepest crater was obtained with the 1.5% ICG concentration; craters showed no evidence of carbonization. The hardness analysis suggested that the laser significantly decreased the enamel hardness surrounding the irradiated zone. In the lased enamel, mean values of hardness decreased 6.16%. The authors concluded that long-term studies will be needed to substantiate the clinical significance of these findings. During the ablation, temperature recordings showed no significant change in temperature at the pulp chamber. Interestingly, without dye, no cavitation occurred, and there was a temperature rise of 3° C to 4° C at the pulp cavity. Apparently, both wavelengths could remove hard tissues, but only with the use of a dye in the 750-nm wavelength case.
In 1995, Rechmann and Hennig26 first reported that the frequency-doubled alexandrite laser (337 nm, 100 nsec, Q-switched) could selectively remove dental calculus without ablating the underlying enamel or cementum. Based on the difference in the absorption between dentin and subgingival calculus, they assumed that the wavelength of the alexandrite laser may be favorable for selective calculus ablation. Their study revealed that the alexandrite laser at fluence of 1 J/cm2 and pulse repetition rate of 55 Hz, under water cooling, could selectively ablate supragingival and subgingival calculus. With these parameters, the alexandrite laser causes no morphological damage to enamel surface or root cementum,16 although extremely slight compositional change (e.g., minimal reduction of amide II band) was detected in the lased cementum by Fourier-transformed infrared (FTIR) spectroscopy.27 Also, during calculus removal, alexandrite lasers could selectively remove unstained microbial plaque.15
Rechmann et al.28 also demonstrated that there was no pulp damage after the removal of calculus with the alexandrite laser at 1.5-6 J/cm2 and 70 Hz (pulse duration 1 µs) with water cooling in dogs. Pilgrim et al.19 strongly support the use of the frequency-doubled alexandrite laser for calculus removal (377 nm, 1 µsec, 70 Hz, water cooling), even though the mechanism of selective ablation has not yet been clarified. The development of this laser for clinical use is widely expected because of its excellent ability to remove calculus selectively from the tooth or root surface without ablating the tooth structure. However, the use of light in the ultraviolet (UV) part of the spectrum is a concern, and further studies are required to demonstrate the safety and effectiveness of this frequency-doubled alexandrite laser in clinical practice and to develop a laser apparatus appropriate for clinical use.29
In endodontics, Jelinkova et al.30 evaluated the effect of the alexandrite laser (0.75 µ; 250 mJ, 1 Hz, 30 pulses) on root canal bacteria reduction. Alexandrite laser radiation could spread through the canal system space and into the surrounding dentinal tissues, with the capability of effectively killing bacteria: 100% of Nocardia asteroides and Micrococcus albus, 60% of Streptococcus sanguinis, and 40% of Lactobacillus species. Temperature rise was not measured. Dostalova et al.31 verified the effect of the frequency-doubled alexandrite laser (375 nm; 1 Hz, spot size 320 µ; number of pulses 200; energy 1 mJ) in root canal cleaning and morphology. SEM revealed that lased samples had open dentinal tubules with no cracks or surface modifications. The study verified that the alexandrite laser could be useful for cleaning the root canal system. Further studies should evaluate temperature increase on the root surface. The best methodology to perform the irradiation on root canal systems should also be considered.
In the field of dental esthetics, Jelinkova et al.32 tested alexandrite lasers (750 nm) with a laser-activated bleaching agent on discolored teeth. The alexandrite laser with a bleaching agent helped to reach the desired tooth shade after shorter exposure time (400 sec) than the neodymium-doped yttrium-aluminum-garnet (Nd:YAG) laser, with no adverse effects. No cracks or essential surface modifications were observed.
Shah and Alster33 have described the removal of amalgam tattoos in mucosa by Q-switched alexandrite lasers (755 nm; 5.5 J/cm2 applied through 3-mm collimated handpiece). Significant lightening of the tattoo was noted after the third treatment. The wavelength of 755 nm has been touted as more advantageous than the 694-nm ruby laser for tattoo removal because it tends to cause less epidermal tissue destruction as a result of decreased absorption of its longer wavelength by epidermal melanin. The slightly longer wavelength of the alexandrite laser allows less melanin absorption and deeper tissue penetration, thereby reducing skin pigment changes and increasing absorption by the actual tattoo pigment.20 As a result, the incidence of unwanted side effects, such as permanent hypopigmentation, is minimized with Q-switched alexandrite laser treatment.33 The features of alexandrite lasers allow rapid treatment sessions with minimal discomfort.20
Although the advantages and technical features of a compact, pulsed, frequency-doubled alexandrite laser system have been studied for enamel/dentin preparation, soft tissue vaporization/coagulation, and sterilization,34 little research is available on dental applications of alexandrite lasers. Additional study is necessary to determine the potential of laser scaling,29 as well as safe protocols for each clinical application. Also, the concern about UV light requires further studies to demonstrate the clinical safety of this laser.35 However, based on current knowledge,20 the alexandrite laser can selectively remove calculus from the tooth or root surface without ablating the tooth structure and seems to be a promising clinical tool.
Photoactivated Disinfection and Microbial Reduction
The temperature increase resulting from high-intensity laser irradiation can cause protein denaturation and can destroy microorganisms, with high decontamination indexes.36 Low-level laser therapy (LLLT) is not capable of increasing tissue temperature,37 and one cannot expect the same antimicrobial effects when LLLT is used as the sole clinical modality.38 Despite this, low-intensity lasers have been studied and introduced clinically for microbial reduction. Their antimicrobial effect is achieved by the association of low-power lasers to extrinsic photosensitizers, which results in highly reactive oxygen species (ROS).39 These cause damage to cell membranes, mitochondria, and DNA,40–42 and microbial destruction is inevitable. This process is called photoactivated disinfection (PAD), also called photodynamic therapy (PDT), photochemotherapy, and lethal photosensitization (see also Chapter 15).
The antimicrobial capacity of PAD has been used to improve microbial reduction during conventional therapy in periodontics, endodontics, restorative dentistry, and implantology.43–46 Viral inactivation and treatment of herpes simplex virus type 1 (HSV-1) have also been reported.47
Photoactivated disinfection presents various advantages over traditional antimicrobial agents. PAD promotes faster microbial killing, and there is no need to maintain higher concentrations of photosensitizer in the infected area, as with antiseptics and antibiotics.48 The main advantage is attributed to its local action; PAD affects microorganisms at the site of photosensitizer deposition exclusively, whereas systemic drugs have action throughout the body.49 Moreover, PAD does not damage or alter adjacent structures, such as periodontal and periapical tissue, even when higher concentrations of photosensitizer and higher energy densities are used.50
For the effective treatment of bacterial infections, it is paramount to have an adequate light source and a photosensitizer capable of binding to the target pathogen, so that photosensitization may occur in either subgingival or superficial oral tissues. The most frequently used light source for photosensitization in dentistry is the low-power laser because it (1) presents a narrow spectral band that enables a more specific interaction with photosensitizers, (2) can be coupled to optical fibers, and (3) does not cause increased tissue temperatures, as observed with polychromatic light sources.51,52 The use of light-emitting diodes (LEDs) has also been reported.53
Several photosensitizers are available for PAD; however, disinfection of oral pathogens generally necessitates the use of cationic-charged photosensitizers, such as toluidine blue, methylene blue, and poly-l-lysine-chlorin-e6 conjugates.54,55 The interaction between photosensitizers and microorganisms occurs within a few minutes, and this period (incubation or preirradiation time) must be respected before laser irradiation begins.39,55
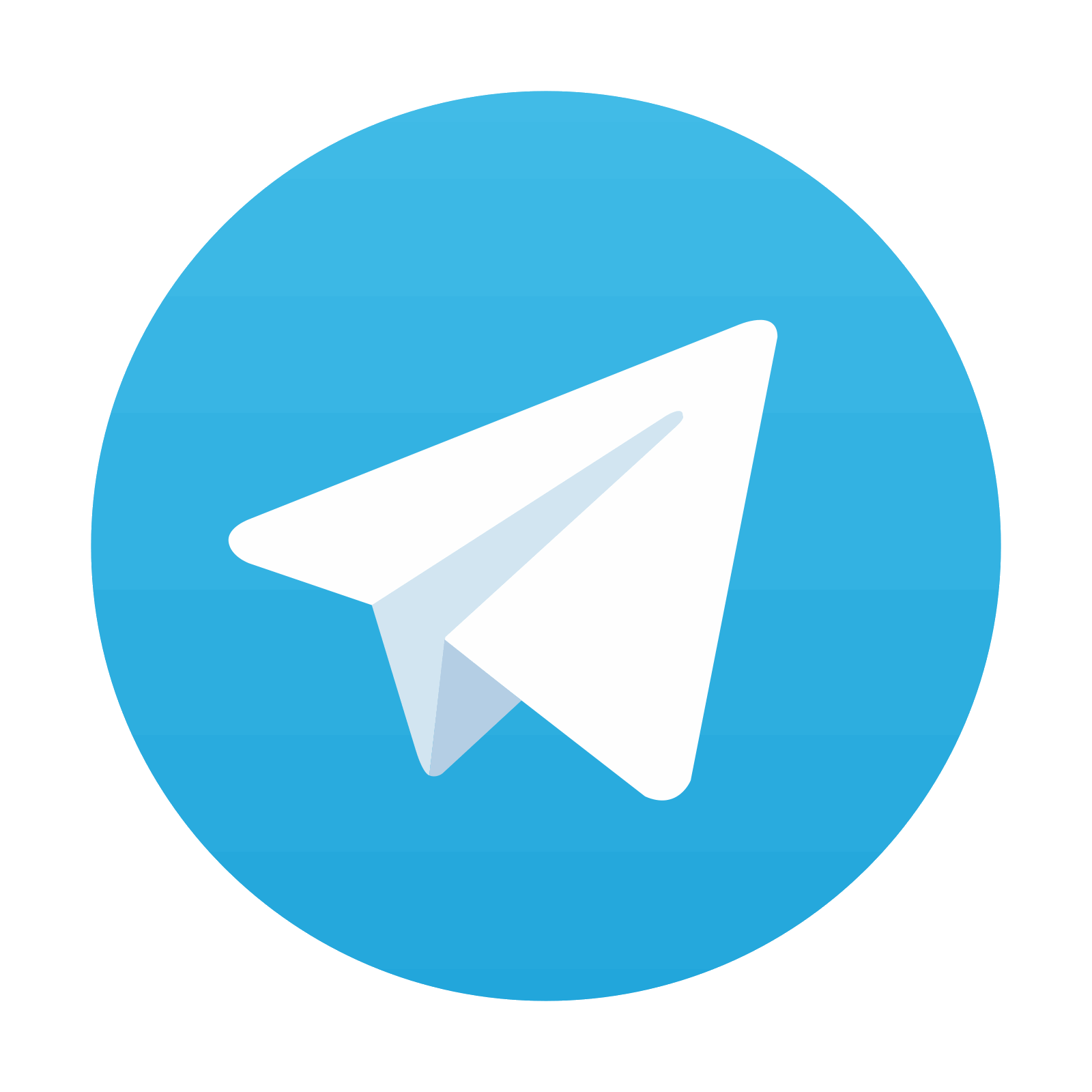
Stay updated, free dental videos. Join our Telegram channel

VIDEdental - Online dental courses
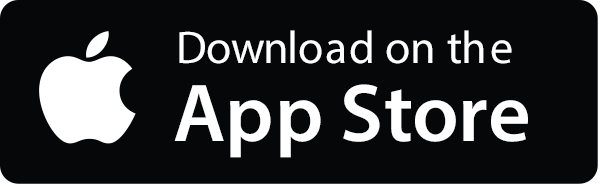
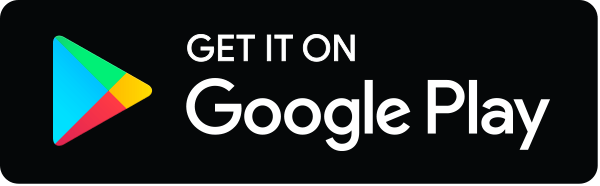