chapter 16 Bone Biology, Osseointegration, and Bone Grafting
Bone is a living tissue that serves two primary functions—structural support and calcium metabolism.1 It has a collagen protein matrix that is impregnated with mineral salts, including calcium phosphate (85%), calcium carbonate (10%), and small quantities of calcium fluoride and magnesium fluoride.2 The collagen protein fibers that form the bone matrix are extremely complex. To maintain normal bone structure, there must be a sufficient quantity of both proteins and minerals. The minerals found in bone are present primarily in the form of hydroxyapatites. Bone mass is an important concept because bone is a mass-efficient structure in which maximal strength is achieved with minimal mass owing to its architecture. Unnecessary bone is lost (e.g., atrophy and bone loss seen in paraplegic patients) through a net loss effect as bone remodeling occurs. Bone also contains small quantities of noncollagen proteins embedded in its mineral matrix. The important family of bone morphogenetic proteins (BMPs) is part of this group.
Bone Biology
A study of bone biology includes elements related to bone cells, metabolism, structure, and repair.
Bone Cells
Three different types of cells—osteoblasts, osteocytes, and osteoclasts—are related to bone metabolism and physiology. The three are closely related to each other and are derived from similar precursors (Figure 16-1).
Osteoblasts, which are associated with the process of osteogenesis, are located in two general areas next to the bone surfaces where they deposit bone matrix. Therefore, they frequently are referred to as endosteal osteoblasts or periosteal osteoblasts. The cytoplasm of osteoblasts is intensely basophilic, which suggests the presence of ribonucleoproteins related to bone matrix protein component synthesis. Fine granules, which can be observed in the cytoplasm, are closely related to the site of active matrix deposit (Figure 16-2).
Osteoclasts are fused monocytes that appear histologically as multinucleated giant cells located in shallow excavations (Howship’s lacunae) along the mineralized surface.3 The cytoplasm of osteoclasts is slightly basophilic and granular, with characteristic vacuoles. Osteoclasts are responsible for bone resorption and form in response to parathyroid hormone. After the process of local bone resorption is complete, osteoclasts disappear, probably by degeneration.
Bone Metabolism
Bone, the primary reservoir of calcium, has a tremendous turnover capability for responding to the metabolic needs of the body and is critical for maintaining a stable serum calcium level.1,2 Because calcium participates in many reactions, it has an essential life support function. It works in conjunction with the lungs and kidneys to help maintain the pH balance of the body through the production of additional phosphates and carbonates, as well as by electrical charge conduction in nerve and muscle, including cardiac muscle. In addition, the metabolic environment is an extremely important component of the biomechanical structure of bone. Bone undergoes continuous turnover in response to metabolic reactions, with the skull and jaws unquestionably affected by this turnover.
Metabolic/hormonal interactions play an important role in the maintenance of bone structure, the most important of which is the linkage of bone resorption to new bone apposition through BMP in normal daily remodeling of bone. Approximately 0.7% of a human skeleton is resorbed daily and is replaced by new, healthy bone. Therefore, a turnover in the entire skeleton occurs approximately every 142 days. When osteoblasts lay down bone, they also secrete BMP into the mineral matrix. This acid-insoluble protein resides there until it is released by osteoclastic resorption. This acid insolubility is an evolutionary mechanism by which the pH of 1 created by osteoclasts is able to dissolve bone mineral without affecting BMP.4 Released BMP then is bound to the cell surface of undifferentiated mesenchymal stem cells, where it causes a membrane signal protein to become activated with high-energy phosphate bonds. This activation, in turn, affects the gene sequence in the nucleus, causing expression of osteoblast differentiation and stimulation of new bone production. A disturbance in this linkage may be the center point of osteoporosis.
Aging and metabolic disease states may reduce the normal turnover process, causing an increase in the mean age of the present bone. This increase may lead to fatigue, bone damage, compromised bone healing, failure to integrate implants, or loss of integration with an implant.5 Thus, it becomes very important for surgeons to realize that a compromised status may not be recognized until the clinician attempts to place implants, or until the implants have been in place for some time.
Bone Structure
The macroscopic structure of bone is a continuum from dense cortical tissue to fine trabecular tissue (Figure 16-3). Between the two ends of this spectrum, no histological difference is seen in the type of bone, only in the relative amount of solid substance present and the geometrical fashion in which it is laid down (the size and number of spaces within it). In most cases, both cortical and trabecular tissues are found at every bone site, but the quantity and distribution of each may vary.
Cortical or compact bone is found in the diaphysis of long bones and on the external surface of flat bones (Figure 16-4). This tissue is organized in bony cylinders consolidated around a central blood vessel (referred to as a Haversian system). Trabecular, spongy, cancellous bone occupies substantial space within the bony tissue that constitutes the medullary cavity of the bone (Figure 16-5). The medullary cavities are filled with marrow: red marrow when there is active production of blood cells or a reserve population of mesenchymal stem cells, and yellow marrow when the cavity has been converted into a site for fat storage with age (Figures 16-6, 16-7).
At the microscopic level, four types of bone are present: woven, composite, lamellar, and bundle. Woven bone plays a principal role during healing. The ability of woven bone to form quickly (at a rate of approximately 30 to 60 m per day) is its main property. However, because woven bone is formed so rapidly, it develops in a disorganized fashion without lamellar architecture or Haversian systems and therefore is soft. As a result, woven bone has low biomechanical strength. Although it often is referred to in the literature as “embryonic bone,” this term is somewhat misleading because all adults have the ability to form this type of bone. Instead, woven bone is referred to as phase I bone during bone healing.6 Although woven bone (phase I bone) is laid down quickly, it normally does not last very long because it is not biomechanically sound. Obligatory resorption and replacement with more mature bone, termed phase II or lamellar bone, occurs.6–8 The term “composite bone” is used to describe the transitional state between woven bone (phase I bone) and lamellar bone (phase II bone). It is a woven bone lattice that is filled with lamellar bone.
Osseous Repair (Bone Modeling and Remodeling)
Bone modeling may be controlled by mechanical factors, as is the case with orthodontic tooth movement, or by growth factors, as is the case with bone healing, bone grafting, and osseointegration. Microstrain (ME) is a method of measuring the load applied to bone as percent deformation of tissue. For example, a load of 200 ME produces a deformation of 0.2% of the tissue. Between the range of 200 and 2,500 ME, there is normal functional response, in which strong bone is produced that is effective in facing increased loads. Atrophy occurs in cases in which the force is low (i.e., less than 200 ME). When the load is between 2,500 and 4,000 ME (i.e., a deformation of 0.25% to 0.4%), hypertrophy occurs, and there is a change in the size of the bone segment.9 The modeling that occurs during hypertrophy is lamellar bone formation. If the load exceeds 4,000 ME, there is a pathological overload, and the modeling that occurs is woven bone formation. In this situation, the bone responds as quickly as possible to meet the excessive load by producing the tissue that can be formed the fastest (i.e., woven bone, which has limited load-bearing capacity).9
Placement of a graft that consists of endosteal osteoblasts and stem cells (from a donor site such as the ileum or tibial head), and that is surrounded by a vascular and cellular tissue bed, creates a recipient site with a biochemistry that is hypoxic (O2 tensions, 3 to 10 mm Hg), acidotic (pH 4.0 to 6.0), and rich in lactate.10 The graft itself contains the osteocompetent cell populations, as well as islands of mineralized cancellous bone, fibrin from blood clotting, and platelets within the clot (Figure 16-8).
Within the graft, the platelets entrapped within the clot degranulate within hours of graft placement, releasing platelet-derived growth factor (PDGF). Therefore, the inherent properties of the wound, particularly the oxygen gradient phenomenon and PDGF, initiate early angiogenesis from surrounding capillaries and mitogenesis of transferred osteocompetent cells11.
Although PDGF seems to be the earliest messenger to stimulate early osteoid formation, PDGF probably is replaced by MDGF and other mesenchymal tissue stimulators from the transforming growth factor-beta family. During the first 3 to 7 days, stem cell populations and endosteal osteoblasts produce only a small amount of osteoid. After the vascular network has been established, osteoid production accelerates, presumably as a result of oxygen and nutrient availability. The initial osteoid that forms develops on the surface of the mineralized cancellous trabeculae from the endosteal osteoblasts. Shortly thereafter, individual osteoid islands develop between cancellous bone trabeculae, presumably from stem cells transferred within the graft. A third source of osteoid production develops from circulating stem cells, which also are attracted to the biochemical environment of the wound.12 These stem cells are postulated to seed the graft and proliferate, thereby contributing to osteoid production.
Throughout the first 3 to 4 weeks, this biochemical and cellular phase of bone regeneration proceeds to coalesce individual osteoid islands, surface osteoid on cancellous trabeculae, and host bone to clinically consolidate the graft. This process uses the fibrin network of the graft as a framework. Normally nonmotile cells, such as osteoblasts, may be somewhat motile via the process of endocytosis along a scaffold such as fibrin. The process of endocytosis is merely the transfer of the cell membrane from the retreating edge of the cell through the cytoplasm as a vesicle to the advancing edge to re-form a cell membrane. This process slowly advances the cell and allows it to secrete its product in the process. In this case, the product is osteoid on the fibrin network. This cellular regeneration phase often is referred to as phase I bone regeneration.6 By the time regeneration is nearly complete (4 to 6 weeks), sufficient osteoid production and mineralization have occurred to permit graft function. Bone at this stage has formed without going through a chondroblastic phase and histologically appears as random cellular bone, which a pathologist would refer to as woven bone.13
Because the amount of bone formed during phase I depends on osteocompetent cell density, donor sites with the highest cancellous trabecular bone areas are chosen. In rank order, it has been shown that the posterior and anterior ileum, tibial plateau, femoral head, and mandibular symphysis are potential donor sites with greater availability of cancellous bone than the calvarium, rib, or fibula.14 In addition, enhanced phase I bone yields are achieved by compacting the graft material. Technically, this enhancement often is accomplished with the use of a bone mill, followed by compaction in a syringe and then further compaction into the graft site using bone-packing instruments.
As was previously stated, the biochemistry of the recipient tissue and the graft itself is largely inherent. However, studies and experience with platelet-rich plasma (PRP) additions to the graft have shown early consolidation and graft mineralization in half the time, with a 15% to 30% improvement in trabecular bone density.12 The concept is that PRP, which is a fibrin clot (also called fibrin glue), is rich in platelets, which, in turn, release PDGF. It has been theorized that this enhanced quantity of PDGF initiates the osteocompetent cell activity more completely than what will inherently occur in the graft and clot milieu alone. Additionally, the enhanced fibrin network created by PRP is believed to enhance osteoconduction throughout the graft, supporting graft consolidation.
The cellular bone regeneration that occurs during phase I is disorganized woven bone that is structurally sound but is not structurally on par with mature bone. The random organization and hypercellular nature of this bone are similar to those seen in a fracture callus. This bone will undergo obligatory resorption and replacement remodeling. Eventually, it will be replaced by phase II bone, which is less cellular, more mineralized, and more structurally organized (Figure 16-9).6,13
As occurs with all bone remodeling, the replacement of phase I bone by phase II bone is initiated by osteoclasts, which are fused mononuclear cells that arrive at the graft site though the newly developed vascular network.3 It has been postulated that these osteoclasts resorb phase I bone in a normal remodeling-replacement cycle. BMP is released during resorption of both the newly formed phase I bone and the nonviable original cancellous trabecular bone. As with normal bone turnover, BMP acts as the link or couple between bone resorption and new bone apposition. Stem cells in the graft from the original transplantation and newly arrived stem cells from local tissues and the circulation respond by osteoblast differentiation and new bone formation. New bone forms as the jaw and graft function, developing in response to the demands placed on it. This bone develops into mature Haversian systems and lamellar bone that is capable of withstanding the normal shear forces placed on the jaw through opening and closing functions, and it tolerates impact compressive forces that are typical of denture-borne and implant-borne prosthetic functions. Histologically, such grafts are involved in long-term remodeling that is consistent with normal skeletal turnover. A periosteum and an endosteum develop as part of this long-term remodeling cycle. Radiographically, the graft takes on the morphology and cortical outlines of the mandible or maxilla over several years.
Osseointegration
Studies of roughened titanium implants indicate a three-phase process of osseointegration: osteophyllic phase, osteoconductive phase, and osteoadaptive phase12.
Osteophyllic Phase
When a roughened surface implant is placed into the cancellous marrow space of the mandible or maxilla, only a small amount of bone from the trabecular bone within the marrow is in contact with the metal surface of the implant. The remaining surface of the implant is exposed to the fibrofatty marrow space. The initial response seen is a migration of osteoblasts and osteoid production to the implant surface. The source of these osteoblasts is surface endosteal osteoblasts of the trabecular bone and the inner surface of the buccal and lingual cortex. It is probable that these cells are responding to the release of BMP from surgical placement of the implant and the initial resorption of bone crushed against the metal surface. The osteophyllic phase lasts for approximately 1 month (Figure 16-10).
Osteoadaptive Phase
The final phase, the osteoadaptive phase, begins approximately 4 months after implant placement, at the same time that the osteoconductive phase ends. It is associated with a steady state (no gain or loss of bone against metal) resorption remodeling sequence that continues even after the implants are exposed and loaded (Figure 16-11).
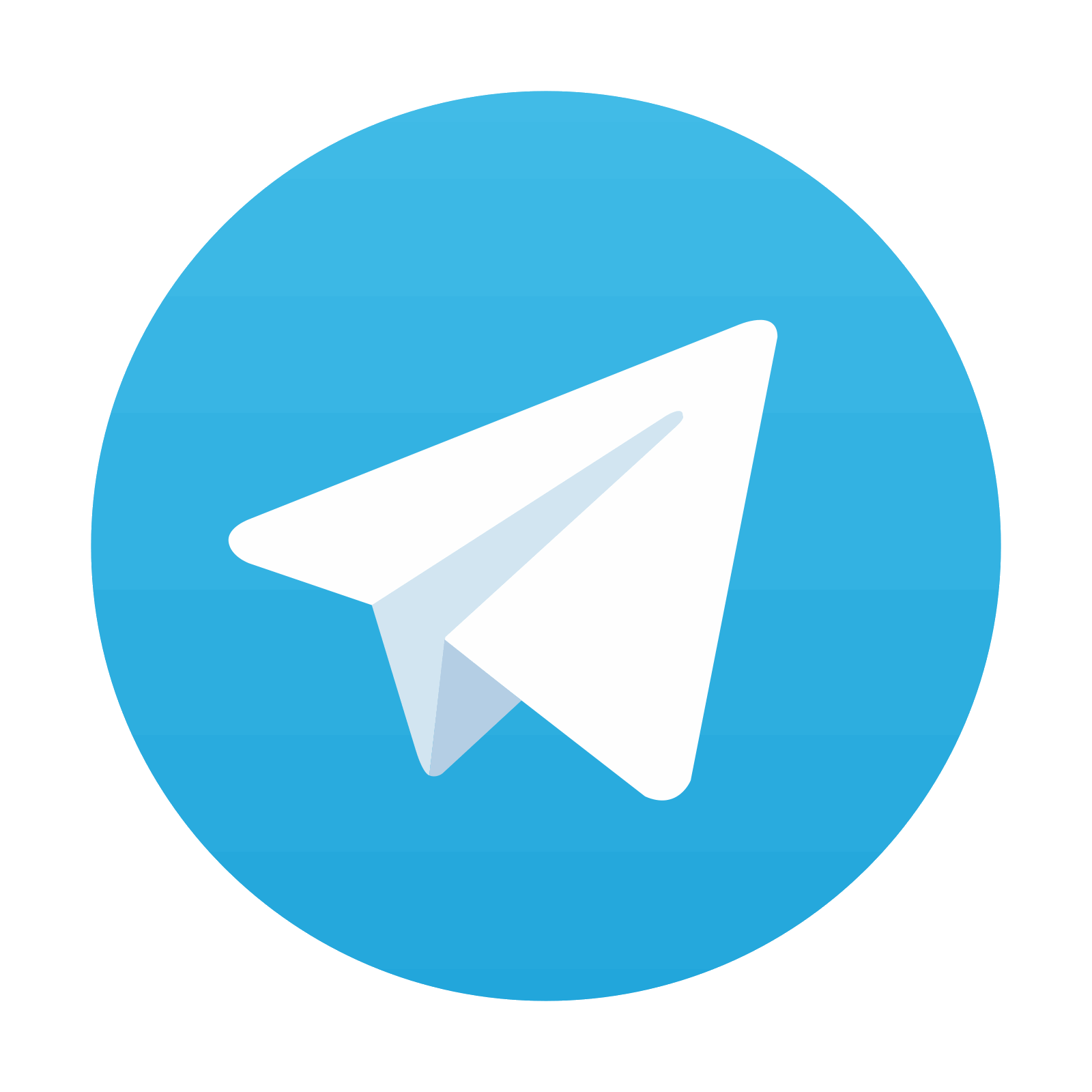
Stay updated, free dental videos. Join our Telegram channel

VIDEdental - Online dental courses
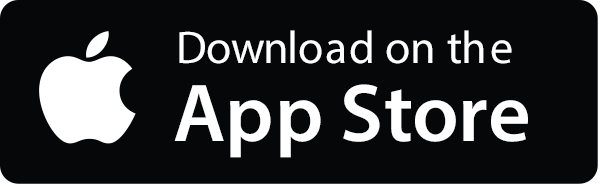
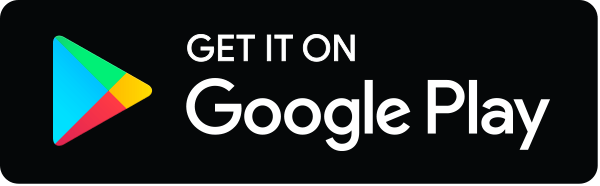