Abstract
Objective
This work measured the amount of bound versus unbound water in completely-demineralized dentin.
Methods
Dentin beams prepared from extracted human teeth were completely demineralized, rinsed and dried to constant mass. They were rehydrated in 41% relative humidity (RH), while gravimetrically measuring their mass increase until the first plateau was reached at 0.064 (vacuum) or 0.116 g H 2 O/g dry mass (Drierite). The specimens were then exposed to 60% RH until attaining the second plateau at 0.220 (vacuum) or 0.191 g H 2 O/g dry mass (Drierite), and subsequently exposed to 99% RH until attaining the third plateau at 0.493 (vacuum) or 0.401 g H 2 O/g dry mass (Drierite).
Results
Exposure of the first layer of bound water to 0% RH for 5 min produced a −0.3% loss of bound water; in the second layer of bound water it caused a −3.3% loss of bound water; in the third layer it caused a −6% loss of bound water. Immersion in 100% ethanol or acetone for 5 min produced a 2.8 and 1.9% loss of bound water from the first layer, respectively; it caused a −4 and −7% loss of bound water in the second layer, respectively; and a −17 and −23% loss of bound water in the third layer. Bound water represented 21–25% of total dentin water. Chemical dehydration of water-saturated dentin with ethanol/acetone for 1 min only removed between 25 and 35% of unbound water, respectively.
Significance
Attempts to remove bound water by evaporation were not very successful. Chemical dehydration with 100% acetone was more successful than 100% ethanol especially the third layer of bound water. Since unbound water represents between 75 and 79% of total matrix water, the more such water can be removed, the more resin can be infiltrated.
1
Introduction
The elegant x-ray diffraction studies of Ramachandran , Bella et al. , Kramer et al. , and the nuclear magnetic resonance (NMR) and other studies by Fullerton et al. and Cameron et al. demonstrated that most proteins are surrounded by bound water. Water distributes electrostatic charges more uniformly than would be possible in the absence of water. Nowhere is water more important than in collagen, the most common structural protein in the body .
The work of Cameron et al. demonstrated that removal of bound water from tendon collagen is very difficult, requiring high vacuum and elevated temperature for days to weeks. However, the relative fraction of total dentin matrix water that is bound versus free has not yet been determined. In resin–dentin bonding, using the wet bonding approach, if too much free water is left in the matrix, application of BisGMA-containing adhesive blends can induce macroscopic phase separation and even nanoscopic phase changes . The use of solvated primers in dentin bonding is an attempt to remove as much free water as possible in a clinically relevant time (30–60 s), to minimize phase changes and provide more volume for resin uptake into resin–dentin interfaces. Residual free water results in increased nanoleakage , water-tree formation , and degradation of matrix collagen .
In adhesive dentistry, dentists acid-etch mineralized dentin with 37% phosphoric acid for 15 s to solubilize apatite crystallites from collagen fibrils so that there is room for solvated adhesive monomers to flow around exposed collagen fibrils, to obtain micromechanical retention of resin composites to the underlying mineralized dentin. Acid-etching uncovers and also activates matrix-bound endogenous proteases of dentin (MMPs and cathepsins) that add water across specific peptide bonds to slowly hydrolyze any collagen fibrils in the hybrid layer that remain uninfiltrated with adhesive resins.
X-ray diffraction studies of the structure of hydrated collagen in bovine tendon revealed that as the water content decreases, the intermolecular spacing decreases to 13 Å and then remains constant even at subzero temperatures. These results indicate that the water that remains within the dehydrated tendon collagen is due to bound water. Increases in water content above 2 g H 2 O/g dry matrix is due to the accumulation of unbound water .
In mineralized dentin, the only free water is located in dentinal tubules and represents about 10 vol% . There may be a low vol% of bound water in mineralized dentin. However, within seconds of acid-etching dentin with 32–37% phosphoric acid, all apatite crystallites are solubilized and extracted by phosphoric acid. The 48 vol% mineral volume is instantly replaced by water that is distributed among tightly-bound, loosely-bound and free water compartments .
Most studies of water bound to collagen have been done on bovine tendon collagen because its collagen fibrils are all arranged parallel to each other . Both Fullerton et al. and Cameron et al. have reported that almost all of the total water in tendon collagen is bound water. Dentin matrix has a very different organization than tendon. Most of the collagen fibrils in dentin are randomly organized. The matrix is penetrated by millions of micro-sized hollow tubules filled with free water . The exact distribution of free versus bound water in demineralized dentin is unknown . Those author identified unfreezable bound water and freezable water in demineralized dentin by differential scanning calorimetry (DSC) and by proton nuclear magnetic resonance ( 1 H NMR). Before resin infiltration of demineralized dentin powder, large 1 H-proton peaks at 4.69 ppm and smaller ones at 0.229 ppm were identified. The peak at 4.69 ppm was assigned to bound water. Although that peak fell to about half its original size after resin infiltration, its persistence indicates that adhesive monomers diffuse over bound water in demineralized dentin during the infiltration phase of dentin bonding . This is very important new information on how resin monomers interact with collagen at the molecular level. Grégoire et al. speculated that residual bound water is needed to prevent the collapse of collagen fibrils in dentin matrices during resin bonding procedures.
Bound water is regarded as structural water . Bound water does not freeze when bulk water freezes . It is unlikely that very much bound water can be removed by evaporation. If bound water represents a significant volume of the intermolecular space between adjacent collagen molecules, there may not be enough room for resin-infiltration . However, Takahashi et al. reported that demineralization of dentin increased the volume of collagen water in dentin, and that HEMA and TEGDMA could equilibrate with that increased volume of water, indicating that such water is not bound, and that there is sufficient room between collagen molecules for free water. Because the ratio of bound to free water has never been measured in dentin matrices, there is uncertainty regarding how much bound versus free water exists in acid-etched dentin.
The purpose of the present study was to quantitate the amount of bound versus free water in demineralized dentin matrices, and to determine how much of that water can be removed by clinically-related bonding procedures within 1–2 min. The test null hypotheses are that the relative amount of bound water does not exceed that of unbound water, that ethanol or acetone does not remove all bound water from demineralized dentin matrices within 1 min, and that ethanol or acetone does not remove all unbound water from demineralized dentin within 1 min.
2
Materials and methods
2.1
Preparation of dentin beams and their complete demineralization
Although our major interest is in the bound and free water content of demineralized dentin surfaces that are only 1–10 μm deep , there are few quantitative analytical methods that can be applied to characterize the bound water content of such microscopic structures. A convenient alternative approach is the macromodel of the hybrid layer .
Unerupted human third molars were collected under a protocol approved by the institutional review board of the Georgia Regents University with informed content. Dentin beams 2 × 1 × 6 mm of mid-coronal dentin were prepared from extracted, unerupted human third molars (18–21 yrs) using an Isomet saw (Buehler Ltd., Lake Bluff, IL, USA). All beams were completely demineralized in 10% phosphoric acid for 16 h at 25 °C with constant tumbling to create macromodels of hybrid layers . Decreases in the modulus of elasticity of dentin beams have been used to demonstrate their complete demineralization . The stiffness of mineralized dentin is 18–20 GPa, while the stiffness of completely demineralized dentin is only 1–2 MPa . After rinsing the completely demineralized beams in running water for 60 min, the beams were placed in water and their modulus of elasticity were determined by 3-point flexure .
2.2
Drying of demineralized dentin under vacuum and elevated temperature
Specimens of completely demineralized dentin beams were dehydrated using the method reported by Cameron et al. . Briefly, the demineralized specimens were placed in a vacuum oven (VWR Scientific, Inc., Atlanta, GA, USA) and subjected to −762 Torr of vacuum (25 °C) created by a vacuum pump until their water content fell to 0.10 g H 2 O/g dry mass. The temperature of the oven was then raised 10 °C/h beginning at 25 °C until it reached 90 °C. The loss of dry mass was followed gravimetrically for 7 days using a Mettler/Toledo AG ultramicrobalance (Model XPS, Greifensee, Switzerland) capable of measuring to the nearest 0.01 mg. Thermal denaturation of the dentin matrix may be prevented if such matrices are partially dried . Previously published differential scanning calorimetry (DSC) studies indicate that the denaturation temperature (T d ) of dentin matrix is 65.5 °C in water-saturated matrices, but 176.1 °C in relatively dry dentin matrices . When the specimens fell to a water content of 0.001 g H 2 O/g dry mass, they were regarded as being absolutely dry.
2.3
Drying of demineralized dentin without vacuum or elevated temperatures
Specimens of completely demineralized dentin beams (2 × 1 × 6 mm) were placed in sealed polyethylene containers half-filled with anhydrous calcium sulfate (Drierite, W.A. Hammond Drierite Co., Xenia, OH, USA) at 37 °C for 3–4 weeks until they reached a constant dry mass of 0.001 g H 2 O/g dry mass.
2.4
Sequential adsortion of bound water from water vapor
The final dry weight of the demineralized dentin beams was 0.001 ± 0.001 g H 2 O/g dry mass. These dry specimens were then slowly rehydrated in a sealed 41 ± 1% relative humidity RH water vapor chamber (Fisher Scientific Traceable Hygrometer, Waltham, MA) at 25 °C. The slow gain in dry mass was measured every 30 min for 12 hrs on a Mettler XPS microbalance, and then every 12 hrs for 48 hrs. When the water content of the beams reached 0.06 g H 2 O/g dry mass, the rate of gain of dry mass abruptly slowed down and reached a plateau after 50 h. This was assumed to indicate that the first layer of bound water had been adsorbed. The specimens were then transferred to a sealed chamber containing a RH of 60% to measure the next increment in bound water adsorption. After about 25 h, the specimen’s mass reached a second plateau of mass gain. Then the specimens were placed in a sealed chamber containing 99% RH and weighed until they reached the third plateau of mass gain.
2.5
Use of low relative humidity to determine if adsorbed water can evaporate
New specimens of demineralized dry dentin were created as described above. When the dry specimens were allowed to reach their first plateau in mass gain, they were transferred to a sealed polyethylene container half-filled with fresh dry Drierite for 5 min at 25 °C and then immediately reweighed to determine if any adsorbed water evaporated into a zero % RH environment.
The experimental specimens were then allowed to adsorb more water until they reached the second plateau in mass gain. Those specimens were then sealed in fresh dry containers at 0% RH for 5 min to determine if any of the adsorbed water could evaporate in 5 min at 25 °C.
After allowing the specimens to reach the third plateau in mass of adsorbed water, they were again sealed into a container of fresh dry Drierite for 5 min to determine if any of the adsorbed water could evaporate from the specimens at 25 °C.
Finally, all specimens were immersed in liquid water at 25 °C for 1 h, gently blot-dried on water-moist Kimwipes (Kimberly-Clark, Roswell, GA, USA) and placed into a sealed container of fresh, dry, Drierite for 5 min to see how much of their fully-hydrated mass could be lost at 25 °C in 5 min in a zero RH environment.
2.6
Use of water-free acetone or ethanol to remove bound water
New specimens of demineralized dry dentin were created as described in the previous section. When they fell to a water content of 0.001 g H 2 O/g dry mass, they were allowed to adsorb water vapor at 25 °C, 41% RH to the first plateau of dry mass. They were then immersed in 100% acetone for 5 min, then removed and the solvents allowed to evaporate for 1 min in 25 °C air to remove residual solvent. Similar experiments were performed for specimens immersed in 100% ethanol for 5 min. These solvents were stored in closed containers containing water-drying polymers (Molecular sieves, catalog number M515-500, Fisher Scientific, Atlanta, GA, USA) to insure that the solvents were dry.
Similar experiments were performed with other completely dry demineralized dentin specimens allowed to adsorb water up the second plateau of dry mass. They were then immersed in 100% acetone or ethanol for 5 min. After removal from the solvents, 1 min was allowed for evaporation of residual solvents before the final dry mass was measured.
The specimens were then exposed to 99% RH and allowed to reach their third plateau of dry mass. They were then immersed in fresh 100% ethanol or 100% acetone for 5 min to determine how much of the bound water could be removed by chemical dehydration. After removal from the solvents, the solvent in the demineralized dentin beams was allowed to evaporate for 1 min prior to remeasurement of their dry mass.
2.7
Statistical analyses
The distribution of bound and unbound water was listed with means ± SDs. The water content was expressed both as mass and as mass of water per mass dry matrix in Table 1 . In Table 2 , the % loss of bound and unbound water after 5 min of evaporation at 0% RH was not normally distributed, so the data were compared by the Kruskal–Wallis test for multiple groups and Dunn’s multiple comparisons test. The percent change in bound water ( Table 3 ) after 5 min exposure to ethanol or acetone was compared using a Kruskal-Wallis test followed by Dunn’s multiple comparisons test. The percent loss of total water from fully hydrated dentin immersed in acetone or ethanol for varying times was compared using a two-way ANOVA (solvent vs. time) ( Table 4 ). Although the data passed normality ( p = 0.785) and equality of variances ( p = 0.530), they showed significant interactions. Multiple comparisons were done using the Least Squares Means test at α = 0.05. The power of that test with an α = 0.05, was 1.00 for solvent, 1.00 for incubation time and 1.00 for incubation time × solvents. When the distributions of bound and unbound water obtained by vacuum/elevated temperature were compared to those obtained using Drierite, the data failed the normality test ( p < 0.05) and the equality of variance test ( p < 0.05). The interactions between bound water and method of drying were significantly ( p < 0.01). Thus, the data were analyzed by least square means. The power of the analyses at a α = 0.05 was 1.0 for plateau, 0.797 for drying method and 1.0 for plateau × drying method.
Specimens dried in vacuum oven | Initial H 2 O content | Dry | Plateau | ||||
---|---|---|---|---|---|---|---|
First | Second | Third | Final H 2 O content | % Recovery | |||
Mass (mg) | 13.74 ± 0.49 | 4.65 ± 0.24 | 4.94 ± 0.29 | 5.67 ± 0.33 | 6.93 ± 0.42 | 10.13 ± 0.7 | – |
g H 2 O/g dry mass | 1.981 ± 0.103 | 0.001 ± 0.001 | 0.064 ± 0.01 | 0.220 ± 0.01 | 0.493 ± 0.04 | 1.171 ± 0.046 | 59.3% |
Specimens dried in drierite | Initial | Dry | Plateau | ||||
---|---|---|---|---|---|---|---|
First | Second | Third | Final | % Recovery | |||
Mass (mg) | 13.37 ± 0.69 | 4.57 ± 0.31 | 5.10 ± 0.34 | 5.43 ± 0.36 | 6.39 ± 0.44 | 12.09 ± 0.98 | – |
g H 2 O/g dry mass | 1.931 ± 0.163 | 0.0008 ± 0.0012 | 0.116 ± 0.003 | 0.191 ± 0.004 | 0.401 ± 0.01 | 1.644 ± 0.094 | 85.0% |
* MD simulation values | 0.000 | 0.0740 | – | 0.637 | – |
* Molecular dynamic simulations—computer simulation of water binding to dry collagen.
Specimen dried in Drierite | % Decrease in mass (%) |
---|---|
First layer bound water | −0.295% ± 0.86 a |
Second layer bound water | −3.27% ± 0.53 a,b |
Third layer bound water | −6.17% ± 1.43 b,c |
Specimens dried in Drierite | Ethanol (%) | Acetone (%) |
---|---|---|
First layer of bound water | 2.80 ± 2.49 a | 1.90 ± 0.06 a |
Second layer of bound water | −3.65 ± 1.15 b | −7.41 ± 0.94 d |
Third layer of bound water | −16.62 ± 2.02 c | −23.30 ± 2.60 e |
Time (min) | Acetone (%) | Ethanol (%) |
---|---|---|
1 | −34.40 ± 3.48 A | −24.65 ± 2.44 B |
2 | −43.29 ± 2.24 C | −30.08 ± 2.09 D |
5 | −58.22 ± 1.99 E | −34.99 ± 4.27 F |
10 | −71.76 ± 2.95 G | −38.38 ± 3.78 F |
2.8
Molecular dynamic simulation of water-binding to collagen
To perform molecular dynamic (MD) simulations of molecular events occurring during resin–dentin bonding, a 3-D model of collagen was constructed using The BuScr. 1.07 , and two of such collagen models were integrated into a simulation box (15.6 Å × 27 Å × 153 Å) to have a hexagonal packing. We employed AMBER99SB-ILDN force field which is known to be the most updated force field for proteins. Water molecules were described by the TIP3P water model . After the energy minimization of the collagen model, MD simulations were performed using GROMACS 4.6.1 . Starting with the initial configuration, the model was energy-minimized to prepare a reasonable starting structure in terms of geometry and solvent orientation, a NVT MD simulation was performed from 10 K to 300 K for 500 ps to stabilize the temperature and pressure of the system, and subsequently, a NPT MD simulation was carried out for 15 ns to equilibrate the system. Then, another NPT MD simulation was performed for 2 ns to collect statistical data for analysis. The first layer of strongly bound water to carbonyl oxygen and amide hydrogens (Ramachandran water bridges) was defined as the waters within the distance of 0–2.25 Å from collagen, and the second layer of less strongly bound water was defined as the waters within the distance of 2.245–3.25 Å from collagen.
2
Materials and methods
2.1
Preparation of dentin beams and their complete demineralization
Although our major interest is in the bound and free water content of demineralized dentin surfaces that are only 1–10 μm deep , there are few quantitative analytical methods that can be applied to characterize the bound water content of such microscopic structures. A convenient alternative approach is the macromodel of the hybrid layer .
Unerupted human third molars were collected under a protocol approved by the institutional review board of the Georgia Regents University with informed content. Dentin beams 2 × 1 × 6 mm of mid-coronal dentin were prepared from extracted, unerupted human third molars (18–21 yrs) using an Isomet saw (Buehler Ltd., Lake Bluff, IL, USA). All beams were completely demineralized in 10% phosphoric acid for 16 h at 25 °C with constant tumbling to create macromodels of hybrid layers . Decreases in the modulus of elasticity of dentin beams have been used to demonstrate their complete demineralization . The stiffness of mineralized dentin is 18–20 GPa, while the stiffness of completely demineralized dentin is only 1–2 MPa . After rinsing the completely demineralized beams in running water for 60 min, the beams were placed in water and their modulus of elasticity were determined by 3-point flexure .
2.2
Drying of demineralized dentin under vacuum and elevated temperature
Specimens of completely demineralized dentin beams were dehydrated using the method reported by Cameron et al. . Briefly, the demineralized specimens were placed in a vacuum oven (VWR Scientific, Inc., Atlanta, GA, USA) and subjected to −762 Torr of vacuum (25 °C) created by a vacuum pump until their water content fell to 0.10 g H 2 O/g dry mass. The temperature of the oven was then raised 10 °C/h beginning at 25 °C until it reached 90 °C. The loss of dry mass was followed gravimetrically for 7 days using a Mettler/Toledo AG ultramicrobalance (Model XPS, Greifensee, Switzerland) capable of measuring to the nearest 0.01 mg. Thermal denaturation of the dentin matrix may be prevented if such matrices are partially dried . Previously published differential scanning calorimetry (DSC) studies indicate that the denaturation temperature (T d ) of dentin matrix is 65.5 °C in water-saturated matrices, but 176.1 °C in relatively dry dentin matrices . When the specimens fell to a water content of 0.001 g H 2 O/g dry mass, they were regarded as being absolutely dry.
2.3
Drying of demineralized dentin without vacuum or elevated temperatures
Specimens of completely demineralized dentin beams (2 × 1 × 6 mm) were placed in sealed polyethylene containers half-filled with anhydrous calcium sulfate (Drierite, W.A. Hammond Drierite Co., Xenia, OH, USA) at 37 °C for 3–4 weeks until they reached a constant dry mass of 0.001 g H 2 O/g dry mass.
2.4
Sequential adsortion of bound water from water vapor
The final dry weight of the demineralized dentin beams was 0.001 ± 0.001 g H 2 O/g dry mass. These dry specimens were then slowly rehydrated in a sealed 41 ± 1% relative humidity RH water vapor chamber (Fisher Scientific Traceable Hygrometer, Waltham, MA) at 25 °C. The slow gain in dry mass was measured every 30 min for 12 hrs on a Mettler XPS microbalance, and then every 12 hrs for 48 hrs. When the water content of the beams reached 0.06 g H 2 O/g dry mass, the rate of gain of dry mass abruptly slowed down and reached a plateau after 50 h. This was assumed to indicate that the first layer of bound water had been adsorbed. The specimens were then transferred to a sealed chamber containing a RH of 60% to measure the next increment in bound water adsorption. After about 25 h, the specimen’s mass reached a second plateau of mass gain. Then the specimens were placed in a sealed chamber containing 99% RH and weighed until they reached the third plateau of mass gain.
2.5
Use of low relative humidity to determine if adsorbed water can evaporate
New specimens of demineralized dry dentin were created as described above. When the dry specimens were allowed to reach their first plateau in mass gain, they were transferred to a sealed polyethylene container half-filled with fresh dry Drierite for 5 min at 25 °C and then immediately reweighed to determine if any adsorbed water evaporated into a zero % RH environment.
The experimental specimens were then allowed to adsorb more water until they reached the second plateau in mass gain. Those specimens were then sealed in fresh dry containers at 0% RH for 5 min to determine if any of the adsorbed water could evaporate in 5 min at 25 °C.
After allowing the specimens to reach the third plateau in mass of adsorbed water, they were again sealed into a container of fresh dry Drierite for 5 min to determine if any of the adsorbed water could evaporate from the specimens at 25 °C.
Finally, all specimens were immersed in liquid water at 25 °C for 1 h, gently blot-dried on water-moist Kimwipes (Kimberly-Clark, Roswell, GA, USA) and placed into a sealed container of fresh, dry, Drierite for 5 min to see how much of their fully-hydrated mass could be lost at 25 °C in 5 min in a zero RH environment.
2.6
Use of water-free acetone or ethanol to remove bound water
New specimens of demineralized dry dentin were created as described in the previous section. When they fell to a water content of 0.001 g H 2 O/g dry mass, they were allowed to adsorb water vapor at 25 °C, 41% RH to the first plateau of dry mass. They were then immersed in 100% acetone for 5 min, then removed and the solvents allowed to evaporate for 1 min in 25 °C air to remove residual solvent. Similar experiments were performed for specimens immersed in 100% ethanol for 5 min. These solvents were stored in closed containers containing water-drying polymers (Molecular sieves, catalog number M515-500, Fisher Scientific, Atlanta, GA, USA) to insure that the solvents were dry.
Similar experiments were performed with other completely dry demineralized dentin specimens allowed to adsorb water up the second plateau of dry mass. They were then immersed in 100% acetone or ethanol for 5 min. After removal from the solvents, 1 min was allowed for evaporation of residual solvents before the final dry mass was measured.
The specimens were then exposed to 99% RH and allowed to reach their third plateau of dry mass. They were then immersed in fresh 100% ethanol or 100% acetone for 5 min to determine how much of the bound water could be removed by chemical dehydration. After removal from the solvents, the solvent in the demineralized dentin beams was allowed to evaporate for 1 min prior to remeasurement of their dry mass.
2.7
Statistical analyses
The distribution of bound and unbound water was listed with means ± SDs. The water content was expressed both as mass and as mass of water per mass dry matrix in Table 1 . In Table 2 , the % loss of bound and unbound water after 5 min of evaporation at 0% RH was not normally distributed, so the data were compared by the Kruskal–Wallis test for multiple groups and Dunn’s multiple comparisons test. The percent change in bound water ( Table 3 ) after 5 min exposure to ethanol or acetone was compared using a Kruskal-Wallis test followed by Dunn’s multiple comparisons test. The percent loss of total water from fully hydrated dentin immersed in acetone or ethanol for varying times was compared using a two-way ANOVA (solvent vs. time) ( Table 4 ). Although the data passed normality ( p = 0.785) and equality of variances ( p = 0.530), they showed significant interactions. Multiple comparisons were done using the Least Squares Means test at α = 0.05. The power of that test with an α = 0.05, was 1.00 for solvent, 1.00 for incubation time and 1.00 for incubation time × solvents. When the distributions of bound and unbound water obtained by vacuum/elevated temperature were compared to those obtained using Drierite, the data failed the normality test ( p < 0.05) and the equality of variance test ( p < 0.05). The interactions between bound water and method of drying were significantly ( p < 0.01). Thus, the data were analyzed by least square means. The power of the analyses at a α = 0.05 was 1.0 for plateau, 0.797 for drying method and 1.0 for plateau × drying method.
Specimens dried in vacuum oven | Initial H 2 O content | Dry | Plateau | ||||
---|---|---|---|---|---|---|---|
First | Second | Third | Final H 2 O content | % Recovery | |||
Mass (mg) | 13.74 ± 0.49 | 4.65 ± 0.24 | 4.94 ± 0.29 | 5.67 ± 0.33 | 6.93 ± 0.42 | 10.13 ± 0.7 | – |
g H 2 O/g dry mass | 1.981 ± 0.103 | 0.001 ± 0.001 | 0.064 ± 0.01 | 0.220 ± 0.01 | 0.493 ± 0.04 | 1.171 ± 0.046 | 59.3% |
Specimens dried in drierite | Initial | Dry | Plateau | ||||
---|---|---|---|---|---|---|---|
First | Second | Third | Final | % Recovery | |||
Mass (mg) | 13.37 ± 0.69 | 4.57 ± 0.31 | 5.10 ± 0.34 | 5.43 ± 0.36 | 6.39 ± 0.44 | 12.09 ± 0.98 | – |
g H 2 O/g dry mass | 1.931 ± 0.163 | 0.0008 ± 0.0012 | 0.116 ± 0.003 | 0.191 ± 0.004 | 0.401 ± 0.01 | 1.644 ± 0.094 | 85.0% |
* MD simulation values | 0.000 | 0.0740 | – | 0.637 | – |
* Molecular dynamic simulations—computer simulation of water binding to dry collagen.
Specimen dried in Drierite | % Decrease in mass (%) |
---|---|
First layer bound water | −0.295% ± 0.86 a |
Second layer bound water | −3.27% ± 0.53 a,b |
Third layer bound water | −6.17% ± 1.43 b,c |
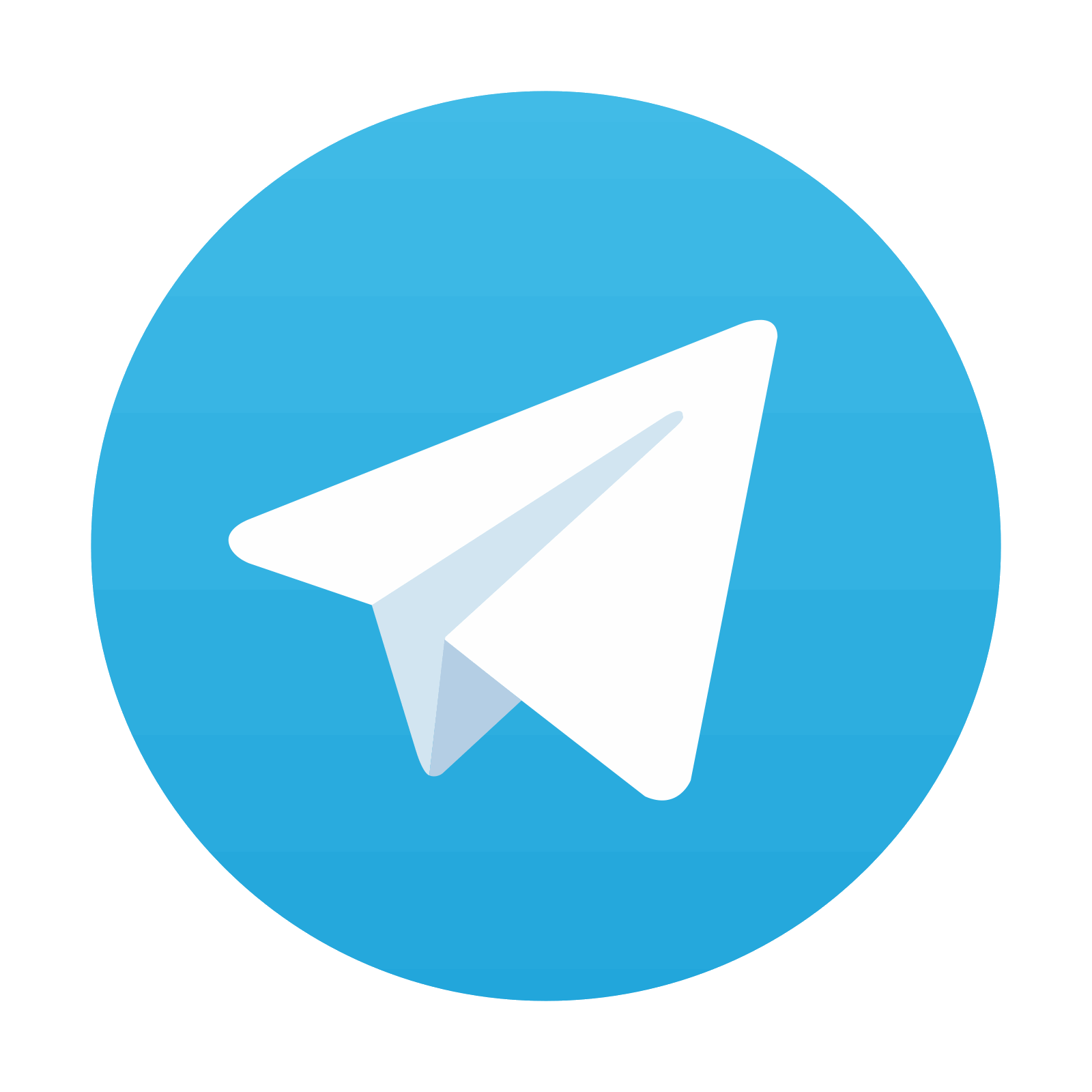
Stay updated, free dental videos. Join our Telegram channel

VIDEdental - Online dental courses
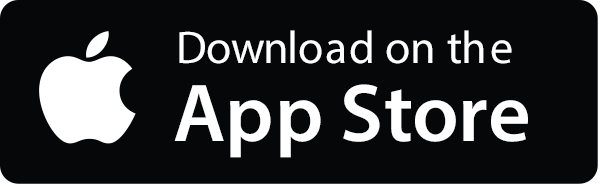
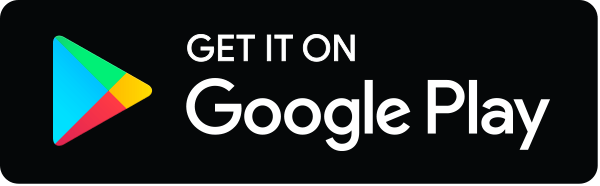
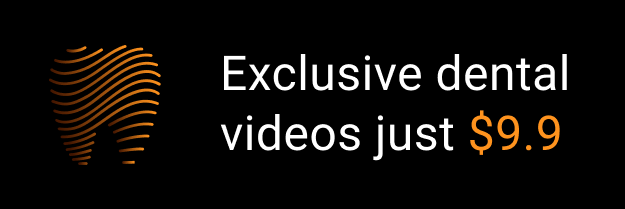