Abstract
The effect of a hypoxia-inducible VEGF-expressing on wound healing in an ischaemic hind leg rat model was evaluated in this study. 180 Wistar rats were assigned randomly to three groups. After ligation of the femoral artery, group 1 received pRTP801-VEGF 165 , group 2 untransfected fibroblasts, group 3 saline; injection was into the subcutaneous tissue, proximal and distal to the artery ligation. Biopsy specimens were obtained on days 3, 5, 7, 14 after implementation. VEGF transgene expression, vessel architecture, the amount and total area of vessel formation were investigated. Results showed a significantly higher level of VEGF protein expression in group 1 compared to group 2 ( P ≤0.001) throughout the investigational period. Group 1 exhibited a significant growth of CD31-positive blood vessels in the subcutaneous tissue on day 14 compared to groups 2 and 3 ( P ≤0.001) (group 1, 62.20± 1.92; group 2, 20.60± 1.67; group 3, 12.40± 1.14). Alpha-SMA-positive staining also showed significant vessel growth in group 1 on day 5 (group 1, 27.00± 1.87; group 2, 7.20± 1.48; group 3, 10.00± 1.73). These results were confirmed in the distal muscle tissue. No significant results were obtained for the proximal muscle tissue. The subcutaneous application of pRTP801-VEGF 165 showed a long-lasting effect, with an increased expression of VEGF over the entire observation period. It appears that the use of fibroblasts transfected with VEGF is a promising way to increase early angiogenesis in ischaemic tissue.
Wound healing is an essential physiological process with the aim of total recovery. To this end, an abundant blood supply is necessary to meet the enormous demand of debridement, fibroblast proliferation, extracellular matrix synthesis, and epithelialization. The induction of localized angiogenesis is of particular interest in the field of plastic and reconstructive surgery to reduce graft loss caused by local ischaemia and necrosis.
Angiogenesis is a complex, continuous process that is controlled by a variety of factors. Vascular endothelial growth factor (VEGF) is a potent direct angiogenic factor that stimulates in vitro endothelial cell migration and activation and in vivo angiogenesis. The availability of oxygen is the strongest regulator of VEGF expression; by decreasing the oxygen partial pressure, the mRNA expression of the gene increases. Uncontrolled expression of VEGF causes unrestrained angiogenesis. Tumour growth relies on the formation of new blood vessels, and in this process, several angiogenic growth factors, including VEGF, are induced. Due to the potential side effects, therapeutic angiogenesis gene therapy should be regulated. The positive effect of in vivo neoangiogenesis stimulation by ex vivo VEGF-transduced fibroblasts has been investigated in previous studies. No potential side effects were detected. Lee et al. examined the potential of a hypoxia-inducible pRTP801-VEGF plasmid and determined strong promoter activity and induction under hypoxia.
Sufficient vascularization of graft tissue and the graft bed is required for a high rate of engraftment. Several preclinical investigations have shown stimulation of perfusion using VEGF. Schultze-Mosgau et al. showed that a reduction in the amount and diameter of capillary structures in pre-irradiated graft tissues is the major cause of graft rejection. With daily topical applications of recombinant endothelial growth factor (rVEGF) to irradiated tissue, an improvement in neovascularization in free vascular graft tissue was detected. Padubidri et al. detected an increased survival rate in an epigastric flap model after injections of VEGF. Histological examination showed that the success was a result of increased capillary concentration.
In this study, we investigated the effects of hypoxia-inducible VEGF gene transfer on neovascularization, VEGF 165 overexpression, and the amount of new blood vessel formation after subcutaneous and intramuscular injections in a rat hind limb model over a period of 14 days, using the RTP801-VEGF 165 promoter.
Methods
Harvest of oral mucosa and in vitro fibroblast culture
A total of 180 male, 3-month-old Wistar rats (weight 500 g; Institute of Laboratory Animal Science, University of Jena, Thuringia, Germany) were used for this study. The animals were kept alone in Makrolon type III cages (Tecniplast, Varese, Italy) at a temperature of 22 ± 0.5 °C, 55% humidity with a 12-h circadian rhythm. The animals received a standard pellet diet (No. 1320; Altromin, Lage, Germany) and fresh water ad libitum . The study was approved by the local authorities (No. 02–002/09). The rats were anaesthetized using techniques described previously. Oral mucosa biopsies measuring 0.5 cm × 1 cm were obtained from the dorso-lateral surface of the tongue of each animal, using previously described techniques. The isolation of the fibroblasts was ensured by the technique of Mueller et al.
Transfection
The first passage fibroblasts were divided randomly into three groups. The cells in group 1 ( n = 60) were transfected with hypoxia-induced pRTP801-VEGF 165 and cultured over another passage of 24 h. The untransfected cells of group 2 ( n = 60) were cultured over another passage of 24 h, and group 3 ( n = 60) had no cells injected. The pRTP801-VEGF 165 plasmid was first investigated by Lee et al. and was kindly provided for this study. The transfections were carried out as described previously. In brief, 5 × 10 7 fibroblasts were resuspended in 100 μl of Nucleofector buffer (Basic Nucleofector Kit for Primary Mammalian Fibroblasts; Amaxa) and nucleofected (Nucleofector I, protocol A024; Amaxa) with 2 μg of the VEGF plasmid. The transfection efficiency was monitored as described previously. Signals were acquired for the forward scatter, side scatter, and the FL1-H channels, excited by the argon laser (488 nm) using standards. A minimum of 10,000 events were acquired per sample. The means and standard deviations (SD) were calculated.
In vivo experiments
Under anaesthesia as described previously, second-passage fibroblasts, either pRTP801-VEGF 165 (group 1) or untransfected fibroblasts (group 2), were injected at a concentration of 5 × 10 7 cells/ml with simultaneous ligation of the femoral artery to provoke hypoxic tissue; group 3 were injected with saline at the time of ligation. The injection was done in the subcutaneous tissue and proximal and distal to the femoral artery ligation. All animals received their own autogenous cells. On days 3, 5, 7, and 14 after surgery, five animals in each group were sacrificed and biopsies were obtained from the area around the injection.
Histology
The tissue specimens were imbedded in Tissue Tek (Sakura Finetek Europe BV, Zoeterwoude, Netherlands) and cut vertically (10 μm) using a microtome. Immunohistochemistry was used to compare new blood vessel formation between the different groups. To avoid unspecified antibody binding, all samples were incubated with 5% rabbit serum (DAKO, Hamburg, Germany) for 30 min. The detection of CD31 was performed with a primary antibody, polyclonal goat VEGF IgG (Santa Cruz Biotechnology, Heidelberg, Germany), at 4 °C for 24 h. The biotinylated polyclonal rabbit anti-goat antibody (DAKO) was used as the secondary antibody at a dilution of 1:100 in Real Antibody Diluent (DAKO) for 60 min at room temperature (RT). To visualize alpha smooth muscle actin (alpha-SMA), a monoclonal mouse anti-human SMA (DAKO) was used as the primary antibody, with incubation at 4 °C for 24 h. Then, the biotinylated rabbit anti-mouse IgG (DAKO) was used at a dilution of 1:100 for 60 min at RT. Three samples per microscope slide were taken, including one negative control (incubation as described, omitting the primary antibody). To stain the secondary antibody, the samples were incubated with streptavidin–horseradish peroxidase (HRP) complex (BioFX Laboratories, Owings Mills, MD, USA) at a dilution of 1:100 for 30 min at RT, followed by incubation with DAB-chromogen (DAKO) for 10 min at RT. To improve the contrast, the nuclei were counterstained with hemalaun.
Histomorphometry
A randomized, systematic sub-sampling method was used. Three randomly selected visual fields per section were digitized at 400× magnification (high-power field, HPF) (Axiovision Zeiss, Oberkochen, Germany). In accordance with Spanholtz et al., each structure with a central lumen surrounded by a continuous layer of CD31- or alpha-SMA-positive cells was counted as a vessel or arteriole. For further validation of the data, the ratio of the total luminal area to the total area of the visual field (as a percentage) was determined using GIMP (GNU Image Manipulation Program, UK).
Real-time polymerase chain reaction (PCR)
A real-time PCR based on SYBR Green (Qiagen, Düsseldorf, Germany) was used to measure the gene expression in the tissue to be examined. The frozen samples were mechanically disturbed for separation in order to determine the amount of RNA using PUREZol RNA Isolation Reagent (Bio-Rad, Munich, Germany). The RNA concentration was defined spectrophotometrically (NanoDrop, Wilmington, DE, USA). The mRNA was quantified using the Rotor-Gene SYBR Green RT-PCR Kit (Qiagen) and Rotor-Gene Q Cycler System (Qiagen). For statistical evaluations, the comparative cycle threshold (Ct) method was used (delta–delta–Ct).
Statistical analysis
All data were recorded as the mean value ± SD. Graphical descriptions were represented with a bar plot. Comparisons between the groups at different time points and among themselves were made using an analysis of variance (ANOVA) test. The P -values were adapted for multiple testing, in accordance with the method of Bonferroni, by multiplying each value with the number of confirmatory tests performed ( n = 3). P -values of <0.05 were considered significant. All calculations were performed using IBM SPSS Statistics for Windows, version 19.0 (IBM Corp., Armonk, NY, USA).
Results
pRTP801-VEGF 165 expression
To examine whether hypoxia-inducible VEGF 165 expression benefits revascularization in early grafts and improves graft survival and function over time, pRTP801-VEGF 165 transfected or non-transfected cells were transplanted. We used a real-time PCR to quantify the expression of VEGF in the hypoxic tissues of the different groups over an investigational period of 14 days.
In the subcutaneous injection area, group 1 (pRTP801-VEGF 165 ) and group 2 (untransfected fibroblasts) showed similar transcription rates at 3 days after ligation of the femoral artery. The expression rates in both groups were similar up to postoperative day 7. Day 14 biopsies from the pRTP801-VEGF 165 transfected group showed a higher gene expression rate compared with the day 14 biopsies from the non-transfected group ( P ≤ 0.007) ( Fig. 1 ).

In the muscular application area distal to the femoral artery ligation, group 1 showed significant VEGF gene expression on day 7 ( P ≤ 0.001) and day 14 ( P ≤ 0.006) compared with group 2 ( Fig. 2 ). In the proximal muscular area, no significant differences between group 1 and group 2 were detected (results not shown).
Neovascularization of hypoxic tissue
We investigated the effect of VEGF overexpression on the neovascularization of tissue with artificially induced hypoxia. For the detection of potential erroneous vessel formation, the results were compared with healthy control tissues. The verifiable CD31- and alpha-SMA-positive vessels showed different results in terms of the application time, region, and group. Visual assessment showed that group 1 (pRTP801-VEGF 165 ) developed the most new blood vessels compared with group 2 (untransfected fibroblasts) and group 3 (saline) ( Figs. 3 and 4 ). Some blood vessels contained erythrocytes in their lumens. CD31-positive blood vessels represent all the vessels detected; alpha-SMA is only stained in smooth muscle.
In the subcutaneous injection area, group 1 showed a significant growth of CD31-positive blood vessels on day 5 ( P ≤ 0.05; group 1, 53.20 ± 1.64; group 2, 17.80 ± 1.89; group 3, 10.80 ± 1.95) and day 14 ( P ≤ 0.05; group 1, 62.20 ± 1.92; group 2, 20.60 ± 1.67; group 3, 12.40 ± 1.14) compared with groups 2 and 3. The vascular lumen was significantly different on day 3 ( P ≤ 0.023) and day 14 ( P ≤ 0.001). The alpha-SMA-positive blood vessels showed significant growth on day 5 (group 1, 27.00 ± 1.87; group 2, 7.20 ± 1.48; group 3, 10.00 ± 1.73); the vascular lumen showed significant differences between group 1 and groups 2 and 3 on day 5 ( P ≤ 0.001) ( Fig. 5 ).
In the distal muscular application area, results for CD-31 positive blood vessels were significant on day 5 (group 1, 53.80 ± 1.68; group 2, 33.80 ± 1.88; group 3, 8.40 ± 1.30) and on day 7 (group 1, 53.80 ± 1.64; group 2, 15.80 ± 1.64; group 3, 9.40 ± 1.14); the vascular lumen of CD-31-positive blood vessels of group 1 showed significant differences on day 5 compared with groups 2 and 3 ( P ≤ 0.001). The alpha-SMA-positive blood vessels showed different results. Significance with regard to the number of alpha-SMA-positive vessels was detected on day 3 (group 1, 26.00 ± 1.81; group 2, 10.80 ± 1.17; group 3, 10.40 ± 1.82) and day 7 (group 1, 25.80 ± 1.70; group 2, 9.70 ± 2.07; group 3, 8.60 ± 2.03). No significant differences in results were found in respect to the vascular lumen ( Fig. 6 ).
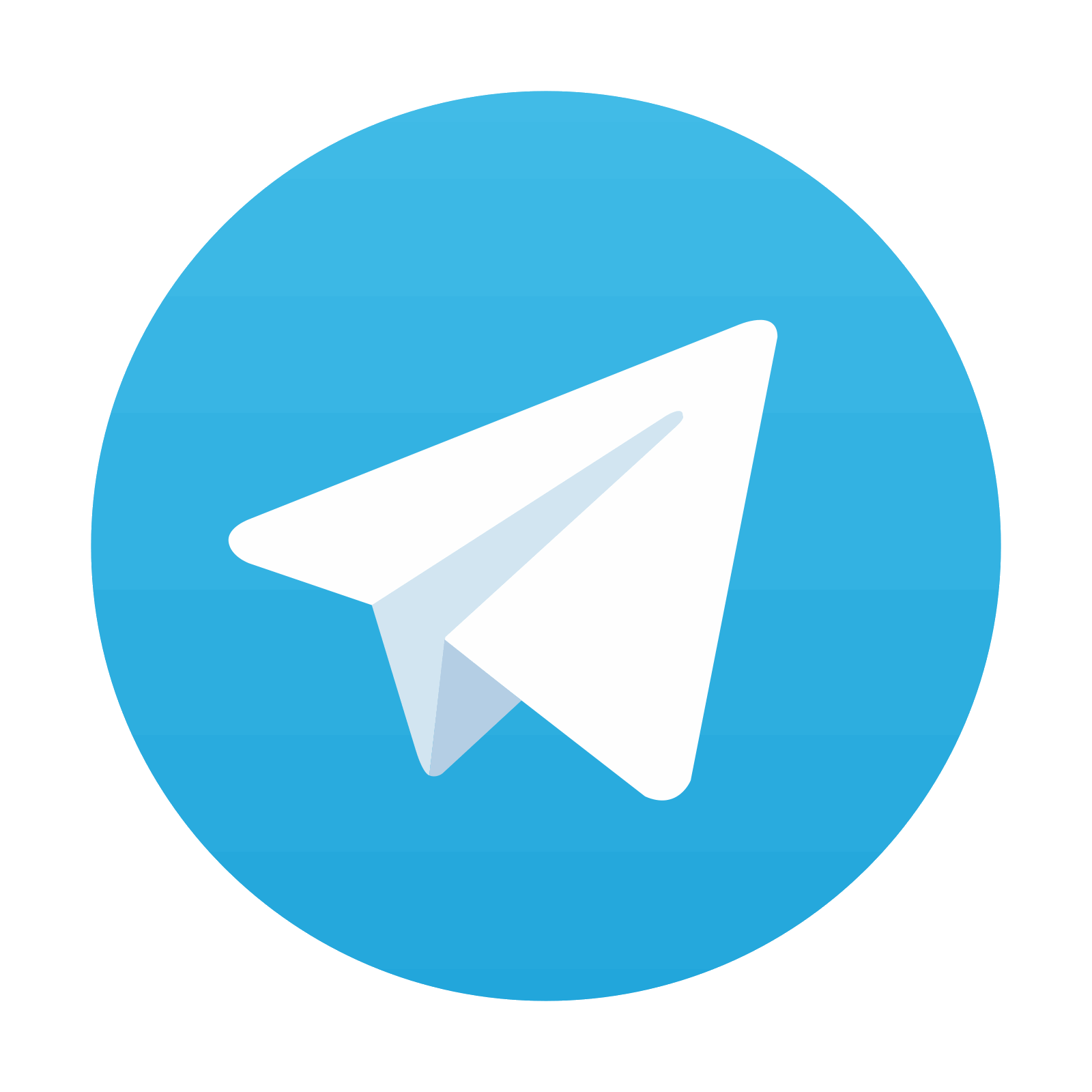
Stay updated, free dental videos. Join our Telegram channel

VIDEdental - Online dental courses
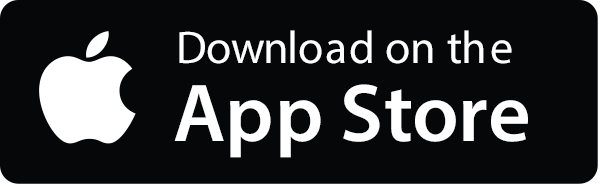
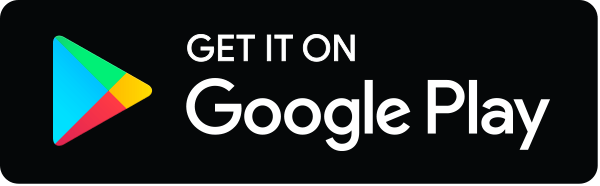
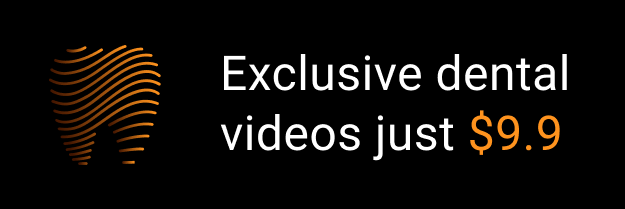