Highlights
- •
We evaluated the impact of alginate- and hyaluronic acid-based hydrogel on SCAP viability.
- •
Hydrogel composition influenced in vitro SCAP viability and metabolic activity.
- •
SCAPs survived in vivo for 1 week.
- •
The appropriate combination of surface and mechanical characteristics dictated SCAP fate.
Abstract
Objective
The goal of the present work was to evaluate in vitro and in vivo the influence of various types and compositions of natural hydrogels on the viability and metabolic activity of SCAPs.
Methods
Two alginate, three hyaluronic-based (Corgel™) hydrogel formulations and Matrigel were characterized for their mechanical, surface and microstructure properties using rheology, X-ray photoelectron spectroscopy and scanning electron microscopy, respectively. A characterized SCAP cell line (RP89 cells) was encapsulated in the different experimental hydrogel formulations. Cells were cultured in vitro , or implanted in cyclosporine treated mice. In vitro cell viability was evaluated using a Live/Dead assay and in vitro cellular metabolic activity was evaluated with a MTS assay. In vivo cell apoptosis was evaluated by a TUNEL test and RP89 cells were identified by human mitochondria immunostaining.
Results
Hydrogel composition influenced their mechanical and surface properties, and their microstructure. In vitro cell viability was above 80% after 2 days but decreased significantly after 7 days (60–40%). Viability at day 7 was the highest in Matrigel (70%) and then in Corgel 1.5 (60%). Metabolic activity increased over time in all the hydrogels, excepted in alginate SLM. SCAPs survived after 1 week in vivo with low apoptosis (<1%). The highest number of RP89 cells was found in Corgel 5.5 (140 cells/mm 2 ).
Significance
Collectively, these data demonstrate that SCAP viability was directly modulated by hydrogel composition and suggest that a commercially available hyaluronic acid-based formulation might be a suitable delivery vehicle for SCAP-based dental pulp regeneration strategies.
1
Introduction
In the past decade, regenerative endodontics have become accepted clinical procedures that aim at regenerating the pulp tissue after necrosis. These procedures have been generally focused on immature teeth , in order to allow for the immature root to develop both in length and in thickness, hoping to reduce the risk of ulterior fracture. Currently, the clinical endodontic regenerative procedure consists of chemical cleaning of the root-canal space using low concentration sodium hypochlorite, with little or no mechanical instrumentation of the root-canal surface. Then, after placement of an inter-appointment medication (either an antibiotic mixture or as suggested more recently calcium hydroxide ), the root canal is rinsed with EDTA, dried, and bleeding is induced by instrumentation beyond the apex . The blood clot formed will then serve as an in situ scaffold for the migration – proliferation – differentiation of progenitor stem cells, originating from the apical papilla, which can remain vital even in the presence of periapical radiolucency . An alternative strategy would consist in directly delivering progenitor stem cells from the apical papilla (SCAPs) into the root canal by incorporating them in hydrogels that would undergo gelification in situ. As mentioned by Murray et al. , more research is needed to develop hydrogels for this specific application, and provide the appropriate conditions for survival, proliferation and differentiation of the SCAPs.
One important feature for the hydrogel to be used in regenerative procedure is to provide appropriate conditions to ensure cell viability by mimicking the microenvironment of target tissues. For soft tissue as for dental pulp regeneration, injectable hydrogels are clinically convenient and seem appropriate in terms of cell differentiation through mechanotransduction . The properties of hydrogels, including chemical composition, stiffness and network porosity and permeability, have been shown to have a significant impact on the survival and differentiation of encapsulated mesenchymal stem cells . Alginates have been used as injectable cell transplantation vehicles due to their biocompatibility, low toxicity, and relatively low cost . Alginate hydrogels are formed by cross-linking the polysaccharide chains by ionic bridges with divalent cations to form a water-insoluble network. Cells may be encapsulated during the cross-linking process to create cell-hydrogel constructs . Natural materials such as hyaluronic acid have also been widely used for tissue regeneration. Hyaluronic acid is a high molecular weight glycosaminoglycan composed of d -glucuronic acid and N-acetyl- d -glucosamine. It interacts with hyaluronic acid-binding proteoglycans as well as collagen . Hyaluronic acid is a natural component of the extracellular matrix (ECM) and cells, including human SCAPs, possess CD44 and other receptors that interact with hyaluronic acid and initiate signaling cascades . Recently, a modified hyaluronic acid-based hydrogel has become commercially available under the name Corgel™. It presents the advantage to combine the potentials of natural and synthetic polymers and also, to be a reliable source of well-characterized hydrogel with controlled properties. This type of hydrogel is indicated for surgical applications and regenerative medicine, but few data are available to date .
Currently, there is no formal comparison of the influence of various injectable hydrogels on the survival of SCAPs. The hydrogel mechanical and chemical properties as well as its network structure interacting with the cells are known to affect the cell viability and behavior . We hypothesized that hydrogel properties will influence SCAP viability and metabolic activity. Hence, the goal of the present work was to evaluate in vitro and in vivo the influence of various types and compositions of alginate and hyaluronic-based hydrogels on the viability and metabolic activity of SCAPs, with special focus on several key characteristics of the hydrogels: mechanical properties, pore size, chemical surface composition. A fully characterized cell line population of SCAPs (RP89 cells) was used in this study .
2
Materials and methods
2.1
Hydrogels formulation
Alginates of different compositions were purchased from Pronova (FMC BioPolymers, NovaMatrix, Philadelphia, USA). Alginate UP MVG (MVG: medium viscosity, >60% guluronate monomer units) and SLM100 (SLM: sterile, low viscosity, >50% mannuronate monomer units) have a molecular weight > 200 kDa and a G/M ratio >1.5 and <1, respectively. Corgel™ hydrogels (Corgel™ BioHydrogel Lifecore kit, Lifecore Biomedical, Chaska, USA) are hyaluronic acid-based hydrogels which form covalent bonds under the action of peroxidase and hydrogen peroxide . Corgel™ polymers with three different tyramine substitution degrees were used (1.5%, 2.8% and 5.5% for Corgel 1.5, Corgel 2.8 and Corgel 5.5, respectively). 0.5% (w/v) Alginate MVG and 1% (w/v) alginate SLM were prepared by dissolving alginate in 3-(N-morpholino)propanesulfonic acid, 4-morpholinepropanesulfonic acid (MOPS) buffer (Sigma–Aldrich, Saint Louis, USA). Hydrogels were formed by addition of a 50 mM CaCl 2 in MOPS (Sigma–Aldrich) solution on the alginate solution. Corgel hydrogels were prepared according to supplier instructions (Lifecore Biomedical). Polymers were dissolved in a horseradish peroxidase (10 U/ml) solution provided by the supplier to obtain 10 mg/ml solutions. Hydrogel formation was initiated by addition of hydrogen peroxide (H 2 O 2 ) with a 1/25 (v/v) (H 2 O 2 gelation solution/Corgel™ polymer solution) ratio. Gelation occurred immediately. High concentration Matrigel™ (BD Biosciences; Bedford, MA) was used as positive control due to its excellent handling properties, easy availability and extensive use for both in vitro and in vivo studies . Matrigel was used as supplied and gelified at 37 °C.
2.2
Analysis of the hydrogel mechanical properties by rheology
Linear viscoelastic properties of hydrogels were investigated by small amplitude oscillatory shear (SAOS) rheology. The storage modulus ( G ′), loss modulus ( G ″) and phase angle ( Φ ) were measured for each hydrogel at 37 °C using a Malvern Kinexus rheometer and rSpace software (Malvern Instruments, Worcestershire, UK). Plates were preheated at 37 °C for 10–15 min before the experiment to compensate for any thermal expansion. No slippage problem was observed due to good adhesion of the hydrogels to the surface of the steel plates. The evolution of G ′, G ″ and Φ upon gel formation were monitored by consecutive frequency sweep (from 100 to 0.1 rad/s) measurements. The SAOS measurements were done in shear-controlled mode with low angular deformation (0.3% strain) to eliminate possible destructive effects of shear on network formation and ensure data acquisition in the linear viscoelastic regime. Measurements were recorded over time, until reaching equilibrium. Conditions were optimized for each hydrogel type. For alginate hydrogels, 50 μl of polymer solution were placed in the center of the fixed-plate. Then, a rotary plate of 8 mm diameter was placed at 1000 μm from the fixed plate. CaCl 2 solution was gently added around the plates to make calcium ions diffuse in the polymer solution, followed by immediate analysis and data acquisition. For Corgel™ hydrogels, 12 μl of H 2 O 2 solution were placed on the center of the fixed plate. Then, 300 μl of polymer solution were added, and a 20 mm-diameter rotary plate was directly placed at 800 μm of the fixed one, followed by immediate analysis and data acquisition. As Corgels™ exhibit lower viscoelastic levels, a 20 mm-diameter parallel plate was used to ensure good signal to noise ratio. Matrigel was measured with a 40 mm-diameter cone and plate geometry with a cone angle of 0.5°.
2.3
Hydrogel surface analysis by X-ray photoelectron spectroscopy (XPS)
To analyze their surface composition, hydrogels were formed (150 μl) as described in Section 2.1 . They were freeze-dried, gently crushed with a spoonbill on two-faces-adhesive tape and placed on a small polyacetal plate. This plate was then placed on the sample-holder. Analyses were done on a Kratos Axis Ultra (Kratos Analytical, UK) spectrometer. A survey spectrum as well as C 1s, O 1s, N 1s, Ca 2p, Na 1s, P 2p, Cl 2p, Si 2p, and S 2p individual spectra were recorded for each sample. Chemical quantification was done with Casa XPS software (Casa Software Ltd., UK). The oxygen/carbon and nitrogen/carbon ratios were used to compare the different hydrogels.
2.4
Incorporation of stem cells from the apical papilla (SCAPs) in hydrogels and microstructure analysis by scanning electron microscopy (SEM)
The stem cells used for this study were isolated from apical papilla of immature permanent teeth purified and fully characterized (RP89 cells) . Cells were grown (37 °C, 5% CO 2 ) in T75 flasks containing 15 ml of Minimum Essential Medium (Sigma–Aldrich) supplemented by 10% bovine serum (Gemini Bio-Products, Sera Laboratories International, Bolney, UK), 1% of l -glutamine (Gemini) and 1% of penicillin and streptomycin (Gemini Bio-Products) (RP89 cell culture medium) until they reach ∼80% confluence. Cells were then subcultured (Accutase, Life Technologies Europe, Gent, BE), counted and re-suspended in the appropriate polymer solution (10 6 cells/ml, unless stated). Hydrogels (100–150 μl) were formed in 96-well plates, by adding the gelation solutions to the polymer-and-cell solutions. Once gelation occurred, hydrogels were equilibrated with PBS, cell culture medium was added on top of the hydrogels and they were incubated at 37 °C, 5% CO 2 . RP89 cells were used at passages 3 and 4.
SEM analysis was performed on RP89 cell-loaded hydrogels (200 μl) prepared as described in Section 2.2 . Hydrogels were formed and cultured 7 days with RP89 cell culture medium at 37 °C, 5% CO 2 . Hydrogel-containing cells were fixed in paraformaldehyde 4%, equilibrated in milliQ water, snap frozen and freeze-dried. Dried samples were cut with a razor blade, so that the internal part of the gel could be exposed to the electron beam. The samples were metalized with a 7 nm chromium layer in a 208HR metalizer (Cressington Scientific instruments, Watford, UK). Images were taken on a microscope JEOL7600F (JEOL Europe, Zaventem, BE), with a FEG filament and a semi-inlens detector. Pore diameter was measured with ImageJ. For each pore, length and width were recorded. For each condition, between 20 and 30 pores were measured and the mean pore diameter was calculated.
2.5
In vitro cell viability
Hydrogel-containing cells (200 μl) were formed in 8 wells Lab-Tek ® (ThermoScientific) and incubated at 37 °C, 5% CO 2 for 2 or 7 days (hydrogel thickness around 2 mm). For each condition, 3 hydrogels containing 10 6 RP89 cells/ml and one control without cell were used. To assess cell viability, a Live/Dead assay was performed. Samples were incubated for 45 min with 10 nM Calcein AM (Life Technologies) and 10 nM EthD-1 (Biotium, Hayward, USA) solutions. Cell viability was then evaluated by confocal imaging (SM700, Zeiss). The samples were imaged from the bottom-up of the hydrogels over a thickness of 405 μm. Three regions (670 × 890 × 405 μm 3 ) randomly chosen were analyzed for each sample ( n = 3 samples/type of hydrogel). Living cells (green) and dead cells (red) were counted using ImageJ software. Cell viability was obtained by dividing the number of living cells by the total amount of cells (Living + dead cells).
2.6
Cellular metabolic activity
RP89 cellular metabolic activity was measured using a CellTiter 96 ® AQ ueous Non-Radioactive Cell Proliferation Assay kit (MTS) (Promega) following supplier instructions. Hydrogels (150 μl) with ( n = 3 samples/hydrogel per experiment) or without cells (2 × 10 6 cells/ml) ( n = 2 samples/hydrogel per experiment) were formed for each condition in a 96-well plate (hydrogel thickness around 4.5 mm). The MTS test was performed at day 2, 3 and 7 ( n = 2 experiments). Optical density (OD) was measured at 492 nm, 2 h after sample incubation with the MTS reagent (Thermo Electron Corporation Multiskan spectrophotometer, ThermoScientific). Once the MTS test was performed at day 2. The samples were washed with PBS and maintained in culture for additional 5 days. Additional measurements were made on day 3 and day 7 in culture as described for day 2. The background optical density measured from the hydrogel without cells (control group) was subtracted from the OD obtained for cell-loaded hydrogels.
2.7
In vivo cell apoptosis
The animal experiments were approved by the ethical committee for animal care of the medical science sector of Université catholique de Louvain. For each condition, 50 μl hydrogels with (2 × 10 6 cells/ml, n = 6) or without cells ( n = 2) were formed in 96-well plates. This volume was chosen to mimic the average volume that could be injected in the cavity created by a pulpotomy performed in human wisdom teeth. Samples were incubated for 2 days prior to implantation and were then placed in peritoneal pockets of NMRI female mice (Janvier, Saint Berthevin, FR). Mice were given 200 μl of anaesthesic solution (18.75 μl Anesketin ® , 25 μl Domitor ® , 156.25 μl PBS). Furthermore 100 μl of Temgesic ® (25 μg/ml) were locally administered. A longitudinal incision was made on the peritoneum. After a ventral incision, the peritoneum was exposed upon tissue dissection. A pocket was created in the peritoneum where the hydrogel containing cells was placed. The pockets were closed with 6/0 Prolene stitches (Ethicon, Johnson & Johnson International, BE). Peritoneum and skin were then stitched. Mice were given 100 μl of Antisedan solution (5 μl Antisedan ® , 95 μl PBS). Mice received 10 mg/kg of cyclosporine (Noeral, Novartis Pharma, Vilvoorde, BE) i.p. one day prior surgery and everyday until the end of the experiment. One week later, samples were retrieved and fixed 24 h in paraformaldehyde 4%. Then, they were placed in agar and processed for paraffin embedding. Samples were sectioned into 10 μm slices (HM 325 Manual Rotary MicrotomeThermoFischer Scientific, Waltham, USA). Collagen was stained by a Masson’s trichrome staining. Cell apoptosis was analyzed by TUNEL (Promega Benelux, Leiden, NL) following supplier instructions. Human cells were detected by immunohistochemistry as previously described against the human mitochondria antigen (HumMit, 1/1000, Millipore, Temecula, USA). Sections were either scanned with a Mirax scan (Zeiss, Zaventem, BE) for TUNEL staining (fluorescence) or a slide scanner SCN400 (Leica Microsystems, Diegem, BE) for HumMit staining (brightfield). The percentage of TUNEL positive cells over analyzed surface was calculated with Axiovision (Zeiss) and the number of HumMit positive cells per mm 2 was counted with ImageJ (NIH, USA) ( n = 4–6 sections/condition).
2.8
Statistical analysis
Statistical analyses were performed using PRISM (GraphPad software, CA, USA) and JMP10 (SAS, NC, USA). Two-way ANOVA with post hoc Bonferroni’s multiple comparison tests were performed with a p -value between 0.05 and 0.001. Error bars represent the standard error of the mean in all figures. Contour plots and linear regression analysis (correlation analysis, p < 0.05) were performed with JMP10.
2
Materials and methods
2.1
Hydrogels formulation
Alginates of different compositions were purchased from Pronova (FMC BioPolymers, NovaMatrix, Philadelphia, USA). Alginate UP MVG (MVG: medium viscosity, >60% guluronate monomer units) and SLM100 (SLM: sterile, low viscosity, >50% mannuronate monomer units) have a molecular weight > 200 kDa and a G/M ratio >1.5 and <1, respectively. Corgel™ hydrogels (Corgel™ BioHydrogel Lifecore kit, Lifecore Biomedical, Chaska, USA) are hyaluronic acid-based hydrogels which form covalent bonds under the action of peroxidase and hydrogen peroxide . Corgel™ polymers with three different tyramine substitution degrees were used (1.5%, 2.8% and 5.5% for Corgel 1.5, Corgel 2.8 and Corgel 5.5, respectively). 0.5% (w/v) Alginate MVG and 1% (w/v) alginate SLM were prepared by dissolving alginate in 3-(N-morpholino)propanesulfonic acid, 4-morpholinepropanesulfonic acid (MOPS) buffer (Sigma–Aldrich, Saint Louis, USA). Hydrogels were formed by addition of a 50 mM CaCl 2 in MOPS (Sigma–Aldrich) solution on the alginate solution. Corgel hydrogels were prepared according to supplier instructions (Lifecore Biomedical). Polymers were dissolved in a horseradish peroxidase (10 U/ml) solution provided by the supplier to obtain 10 mg/ml solutions. Hydrogel formation was initiated by addition of hydrogen peroxide (H 2 O 2 ) with a 1/25 (v/v) (H 2 O 2 gelation solution/Corgel™ polymer solution) ratio. Gelation occurred immediately. High concentration Matrigel™ (BD Biosciences; Bedford, MA) was used as positive control due to its excellent handling properties, easy availability and extensive use for both in vitro and in vivo studies . Matrigel was used as supplied and gelified at 37 °C.
2.2
Analysis of the hydrogel mechanical properties by rheology
Linear viscoelastic properties of hydrogels were investigated by small amplitude oscillatory shear (SAOS) rheology. The storage modulus ( G ′), loss modulus ( G ″) and phase angle ( Φ ) were measured for each hydrogel at 37 °C using a Malvern Kinexus rheometer and rSpace software (Malvern Instruments, Worcestershire, UK). Plates were preheated at 37 °C for 10–15 min before the experiment to compensate for any thermal expansion. No slippage problem was observed due to good adhesion of the hydrogels to the surface of the steel plates. The evolution of G ′, G ″ and Φ upon gel formation were monitored by consecutive frequency sweep (from 100 to 0.1 rad/s) measurements. The SAOS measurements were done in shear-controlled mode with low angular deformation (0.3% strain) to eliminate possible destructive effects of shear on network formation and ensure data acquisition in the linear viscoelastic regime. Measurements were recorded over time, until reaching equilibrium. Conditions were optimized for each hydrogel type. For alginate hydrogels, 50 μl of polymer solution were placed in the center of the fixed-plate. Then, a rotary plate of 8 mm diameter was placed at 1000 μm from the fixed plate. CaCl 2 solution was gently added around the plates to make calcium ions diffuse in the polymer solution, followed by immediate analysis and data acquisition. For Corgel™ hydrogels, 12 μl of H 2 O 2 solution were placed on the center of the fixed plate. Then, 300 μl of polymer solution were added, and a 20 mm-diameter rotary plate was directly placed at 800 μm of the fixed one, followed by immediate analysis and data acquisition. As Corgels™ exhibit lower viscoelastic levels, a 20 mm-diameter parallel plate was used to ensure good signal to noise ratio. Matrigel was measured with a 40 mm-diameter cone and plate geometry with a cone angle of 0.5°.
2.3
Hydrogel surface analysis by X-ray photoelectron spectroscopy (XPS)
To analyze their surface composition, hydrogels were formed (150 μl) as described in Section 2.1 . They were freeze-dried, gently crushed with a spoonbill on two-faces-adhesive tape and placed on a small polyacetal plate. This plate was then placed on the sample-holder. Analyses were done on a Kratos Axis Ultra (Kratos Analytical, UK) spectrometer. A survey spectrum as well as C 1s, O 1s, N 1s, Ca 2p, Na 1s, P 2p, Cl 2p, Si 2p, and S 2p individual spectra were recorded for each sample. Chemical quantification was done with Casa XPS software (Casa Software Ltd., UK). The oxygen/carbon and nitrogen/carbon ratios were used to compare the different hydrogels.
2.4
Incorporation of stem cells from the apical papilla (SCAPs) in hydrogels and microstructure analysis by scanning electron microscopy (SEM)
The stem cells used for this study were isolated from apical papilla of immature permanent teeth purified and fully characterized (RP89 cells) . Cells were grown (37 °C, 5% CO 2 ) in T75 flasks containing 15 ml of Minimum Essential Medium (Sigma–Aldrich) supplemented by 10% bovine serum (Gemini Bio-Products, Sera Laboratories International, Bolney, UK), 1% of l -glutamine (Gemini) and 1% of penicillin and streptomycin (Gemini Bio-Products) (RP89 cell culture medium) until they reach ∼80% confluence. Cells were then subcultured (Accutase, Life Technologies Europe, Gent, BE), counted and re-suspended in the appropriate polymer solution (10 6 cells/ml, unless stated). Hydrogels (100–150 μl) were formed in 96-well plates, by adding the gelation solutions to the polymer-and-cell solutions. Once gelation occurred, hydrogels were equilibrated with PBS, cell culture medium was added on top of the hydrogels and they were incubated at 37 °C, 5% CO 2 . RP89 cells were used at passages 3 and 4.
SEM analysis was performed on RP89 cell-loaded hydrogels (200 μl) prepared as described in Section 2.2 . Hydrogels were formed and cultured 7 days with RP89 cell culture medium at 37 °C, 5% CO 2 . Hydrogel-containing cells were fixed in paraformaldehyde 4%, equilibrated in milliQ water, snap frozen and freeze-dried. Dried samples were cut with a razor blade, so that the internal part of the gel could be exposed to the electron beam. The samples were metalized with a 7 nm chromium layer in a 208HR metalizer (Cressington Scientific instruments, Watford, UK). Images were taken on a microscope JEOL7600F (JEOL Europe, Zaventem, BE), with a FEG filament and a semi-inlens detector. Pore diameter was measured with ImageJ. For each pore, length and width were recorded. For each condition, between 20 and 30 pores were measured and the mean pore diameter was calculated.
2.5
In vitro cell viability
Hydrogel-containing cells (200 μl) were formed in 8 wells Lab-Tek ® (ThermoScientific) and incubated at 37 °C, 5% CO 2 for 2 or 7 days (hydrogel thickness around 2 mm). For each condition, 3 hydrogels containing 10 6 RP89 cells/ml and one control without cell were used. To assess cell viability, a Live/Dead assay was performed. Samples were incubated for 45 min with 10 nM Calcein AM (Life Technologies) and 10 nM EthD-1 (Biotium, Hayward, USA) solutions. Cell viability was then evaluated by confocal imaging (SM700, Zeiss). The samples were imaged from the bottom-up of the hydrogels over a thickness of 405 μm. Three regions (670 × 890 × 405 μm 3 ) randomly chosen were analyzed for each sample ( n = 3 samples/type of hydrogel). Living cells (green) and dead cells (red) were counted using ImageJ software. Cell viability was obtained by dividing the number of living cells by the total amount of cells (Living + dead cells).
2.6
Cellular metabolic activity
RP89 cellular metabolic activity was measured using a CellTiter 96 ® AQ ueous Non-Radioactive Cell Proliferation Assay kit (MTS) (Promega) following supplier instructions. Hydrogels (150 μl) with ( n = 3 samples/hydrogel per experiment) or without cells (2 × 10 6 cells/ml) ( n = 2 samples/hydrogel per experiment) were formed for each condition in a 96-well plate (hydrogel thickness around 4.5 mm). The MTS test was performed at day 2, 3 and 7 ( n = 2 experiments). Optical density (OD) was measured at 492 nm, 2 h after sample incubation with the MTS reagent (Thermo Electron Corporation Multiskan spectrophotometer, ThermoScientific). Once the MTS test was performed at day 2. The samples were washed with PBS and maintained in culture for additional 5 days. Additional measurements were made on day 3 and day 7 in culture as described for day 2. The background optical density measured from the hydrogel without cells (control group) was subtracted from the OD obtained for cell-loaded hydrogels.
2.7
In vivo cell apoptosis
The animal experiments were approved by the ethical committee for animal care of the medical science sector of Université catholique de Louvain. For each condition, 50 μl hydrogels with (2 × 10 6 cells/ml, n = 6) or without cells ( n = 2) were formed in 96-well plates. This volume was chosen to mimic the average volume that could be injected in the cavity created by a pulpotomy performed in human wisdom teeth. Samples were incubated for 2 days prior to implantation and were then placed in peritoneal pockets of NMRI female mice (Janvier, Saint Berthevin, FR). Mice were given 200 μl of anaesthesic solution (18.75 μl Anesketin ® , 25 μl Domitor ® , 156.25 μl PBS). Furthermore 100 μl of Temgesic ® (25 μg/ml) were locally administered. A longitudinal incision was made on the peritoneum. After a ventral incision, the peritoneum was exposed upon tissue dissection. A pocket was created in the peritoneum where the hydrogel containing cells was placed. The pockets were closed with 6/0 Prolene stitches (Ethicon, Johnson & Johnson International, BE). Peritoneum and skin were then stitched. Mice were given 100 μl of Antisedan solution (5 μl Antisedan ® , 95 μl PBS). Mice received 10 mg/kg of cyclosporine (Noeral, Novartis Pharma, Vilvoorde, BE) i.p. one day prior surgery and everyday until the end of the experiment. One week later, samples were retrieved and fixed 24 h in paraformaldehyde 4%. Then, they were placed in agar and processed for paraffin embedding. Samples were sectioned into 10 μm slices (HM 325 Manual Rotary MicrotomeThermoFischer Scientific, Waltham, USA). Collagen was stained by a Masson’s trichrome staining. Cell apoptosis was analyzed by TUNEL (Promega Benelux, Leiden, NL) following supplier instructions. Human cells were detected by immunohistochemistry as previously described against the human mitochondria antigen (HumMit, 1/1000, Millipore, Temecula, USA). Sections were either scanned with a Mirax scan (Zeiss, Zaventem, BE) for TUNEL staining (fluorescence) or a slide scanner SCN400 (Leica Microsystems, Diegem, BE) for HumMit staining (brightfield). The percentage of TUNEL positive cells over analyzed surface was calculated with Axiovision (Zeiss) and the number of HumMit positive cells per mm 2 was counted with ImageJ (NIH, USA) ( n = 4–6 sections/condition).
2.8
Statistical analysis
Statistical analyses were performed using PRISM (GraphPad software, CA, USA) and JMP10 (SAS, NC, USA). Two-way ANOVA with post hoc Bonferroni’s multiple comparison tests were performed with a p -value between 0.05 and 0.001. Error bars represent the standard error of the mean in all figures. Contour plots and linear regression analysis (correlation analysis, p < 0.05) were performed with JMP10.
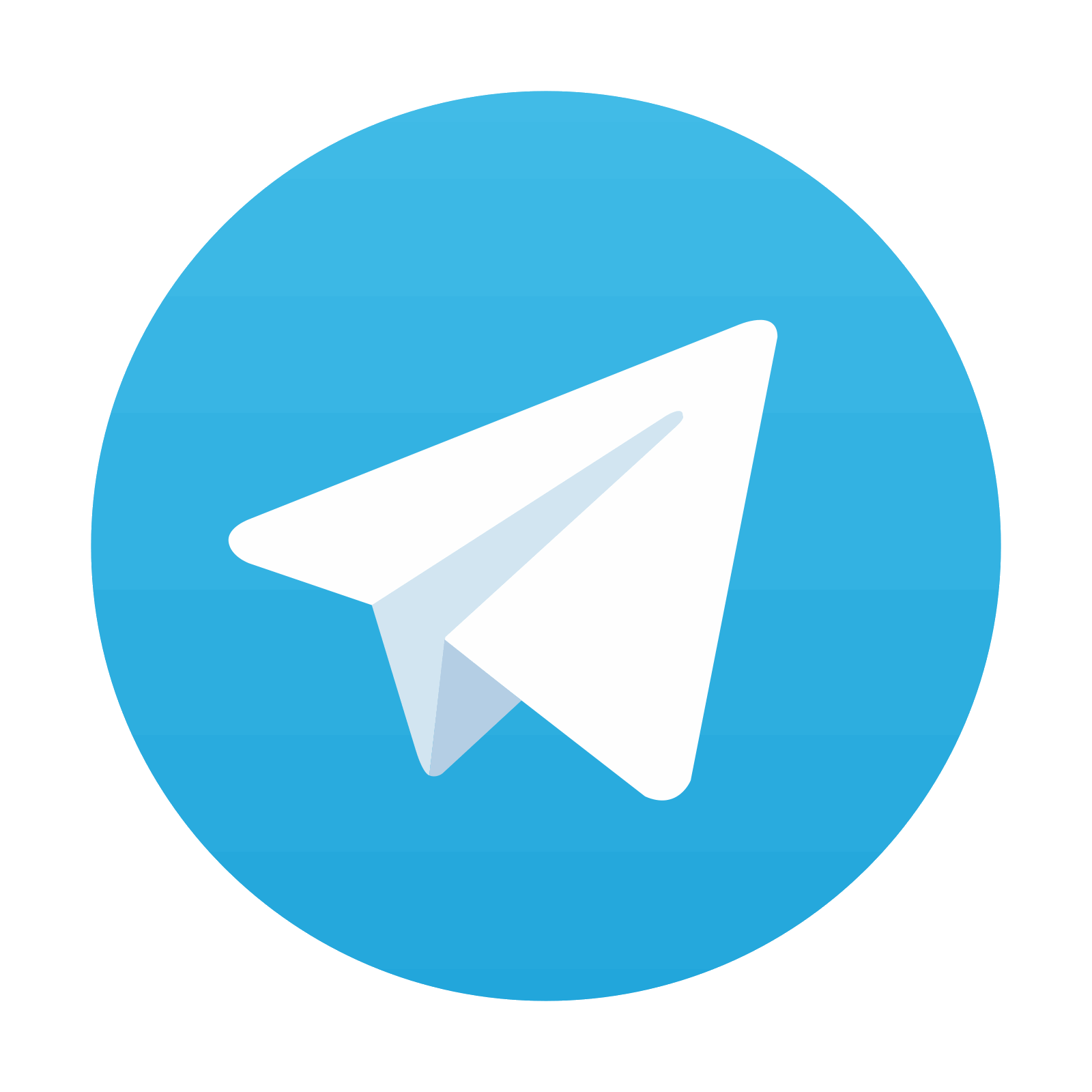
Stay updated, free dental videos. Join our Telegram channel

VIDEdental - Online dental courses
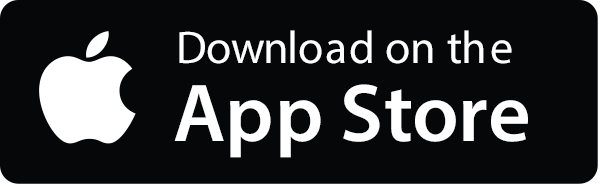
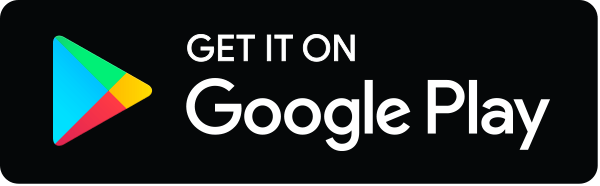