22
The Role of Biomedical Engineers in the Design and Manufacture of Orthodontic Appliances
William A. Brantley1 and Theodore Eliades2
1 Division of Restorative and Prosthetic Dentistry, College of Dentistry, The Ohio State University, Columbus, OH, USA
2 Clinic of Orthodontics and Pediatric Dentistry, Center of Dental Medicine, University of Zurich, Zurich, Switzerland
The broad scope of modern biomedical engineering (Box 22.1) is based on integration of engineering principles with biological sciences to develop devices, materials, and procedures that improve the health of people. To seek past examples of collaboration between biomedical engineers and orthodontists, a search of the biomedical literature using PubMed and the search terms “orthodontic appliances” and “biomedical engineering” was performed and revealed 99 publications since 1971 in the areas of archwire–bracket friction; archwires; force measurements, including degradation of force‐delivery modules; orthodontic appliances, including use of finite element analysis; and miscellaneous subjects, such as Streptococcus mutans and polymerase chain reaction (PCR), allergic reactions to materials, and mechanical behavior of the periodontal ligament. Examination of these publications revealed that the authors typically had biomedical engineering and other engineering affiliations, which accounted for the indexing of the articles by PubMed, although there was generally no specific mention in the articles about the applications of biomedical engineering principles. While these publications are only a small subset of the many published articles in these areas, they nonetheless provide excellent examples of how biomedical engineering principles have been applied in the past to orthodontic appliances. The first part of this chapter will summarize important past research activities involving collaborations between biomedical engineers and orthodontists, and the second part will describe current areas of research and potential future applications where there are highly promising new opportunities for such collaboration.
Past research activities
Archwire–bracket friction
The principal past research to understand the scientific basis of archwire–bracket friction and its applications to clinical orthodontic treatment was performed by the late Professor Robert Kusy and his group of graduate students. Kusy was jointly appointed to the Department of Biomedical Engineering of the School of Medicine and in the School of Dentistry at the University of North Carolina at Chapel Hill.
Kusy’s initial study (Kusy et al., 1988) investigated the effects of surface roughness measured by laser specular reflectance (Figure 22.1) on the coefficients of static and dynamic friction in the dry state for model orthodontic systems that employed metallic archwires, lapped stainless steel, and as‐received polycrystalline alumina surfaces to simulate brackets (Kusy and Whitley, 1990a). All–stainless steel couples of archwire and contacting surface had lower kinetic coefficients of friction than stainless steel–alumina couples, and beta‐titanium had the highest coefficient of friction among archwires, although nickel‐titanium archwires had the roughest surfaces. Scanning electron microscope (SEM) observations using energy‐dispersive x‐ray analyses revealed adhesion of beta‐titanium archwires to stainless steel flat surfaces and abrasion of beta‐titanium archwires by flat alumina surfaces that were microscopically rough due to the sharp facets of the fine grains of the material.
These pioneering studies with model systems were followed by an extensive series of investigations that evolved as new archwire and bracket products were introduced. Dry state friction was measured for archwires in stainless steel and polycrystalline alumina bracket slots by Kusy and Whitley (1990b). The resistance to sliding of self‐ligating brackets and conventional stainless steel twin brackets for second‐order angulation in the dry and wet (saliva) states was studied by Thorstenson and Kusy (2001), followed by evaluation of the sliding resistance of stainless steel archwires in self‐ligating brackets with passive slides and active clips (Thorstenson and Kusy, 2002a). Thorstenson and Kusy (2002b) then examined four designs of self‐ligating brackets coupled with four different nickel‐titanium archwires varying in size and nickel‐titanium phase (Brantley, 2001). In subsequent studies, Thorstenson and Kusy (2003a) first compared the resistance to sliding for polycrystalline alumina brackets and polycarbonate brackets, with and without stainless steel inserts with control stainless steel brackets with stainless steel archwires, and then (Thorstenson and Kusy, 2003b, 2004) investigated the resistance to sliding for stainless steel archwires coupled to conventional stainless steel brackets and new bracket designs. Figures 22.2 and 22.3 (Rucker and Kusy, 2002a) illustrate the principles, schematic apparatus, and typical results for friction measurements. This study compared the resistance to sliding, in dry and wet (saliva) conditions, of multistrand stainless steel, single‐strand stainless steel, and nickel‐titanium archwires. The coefficients of binding were not affected by saliva but were proportional to wire stiffness, and differences in the kinetic coefficients of friction for stainless steel and nickel‐titanium archwires became unimportant shortly after binding occurred.
In the final two studies of archwire–bracket friction from the Kusy laboratory, a more clinically oriented approach was employed to compare four conventional and four self‐ligating brackets. Standardized archwires were drawn through quadrants of typodont models in dry and wet (saliva) states, and the drawing forces were measured for friction evaluation (Henao and Kusy, 2004). A small archwire simulated the earlier treatment stage, and two larger archwires simulated later stages; typodonts had quadrants with progressively increasing malocclusions. In their following study (Henao and Kusy, 2005) the same methodology was used to seek the optimal archwire–bracket system, using archwires and bracket designs from four manufacturers.

Figure 22.1 Schematic measurement of archwire surface roughness using laser reflectance spectroscopy.
Reproduced with permission from Kusy et al. (2004) / Elsevier.

Figure 22.2 Principles for measurement of archwire–bracket friction, showing normal force (N) applied to multistrand archwire, drawing force (P), distance of movement (δ), and relationships of static and kinetic force to resistance to sliding (RS).
Reproduced with permission from Rucker and Kusy (2002a) / Elsevier.

Figure 22.3 Schematic illustration of drawing force–distance data, showing determination of static and kinetic coefficients of friction (μs‐FR and μk‐FR).
Reproduced with permission from Rucker and Kusy (2002a) / Elsevier.
The foregoing laboratory studies by the Kusy research group provided considerable fundamental understanding about the resistance to sliding for commercial archwires in commercial conventional and self‐ligating brackets, along with the role of the critical angle for binding, the consequences of using inserts in brackets, the results from novel bracket designs, and use of different archwire materials and designs. Such information in principle provides the orthodontist with the ability to select the optimum archwires and brackets for a scientific approach to different patient treatment cases.
There is a prolific literature in this area in which biomedical engineers were not generally involved, with over 300 articles cited by PubMed, beginning with Andreasen and colleagues in 1969. The articles by the Kusy research group were selected to illustrate past interactions between biomedical engineers and orthodontists in the investigation of archwire–bracket friction.
In a review article, Burrow (2009) comments that clinical studies indicate a minimal role for archwire–bracket sliding friction in bodily tooth movement, which instead involves successive steps of archwire binding by the bracket and its release. He also notes that clinical studies to date do not indicate that treatment time is reduced with self‐ligating brackets because of lower friction. This important article stimulated several responses and replies in the Readers’ Forum of the American Journal of Orthodontics and Dentofacial Orthopedics (July and December 2009). The reader is also directed to a classic article by Tidy (1989) on the in vitro measurement of frictional forces in fixed orthodontic appliances, and a later article by Loftus et al. (1999) presenting an in vitro dentoalveolar model for measurement of frictional forces. Reznikov et al. (2010) described a new system for measuring friction in vitro in a study that investigated stainless steel archwires and self‐ligating brackets. These authors found that under certain clinical situations a firm passive bracket clip can adversely affect friction. However, none of these four pieces of research involved collaboration between orthodontists and biomedical engineers. New collaborations are needed for further progress in fundamental understanding of the sliding of different types of archwires in the wide variety of commercially available brackets, and how clinical treatment is affected by the complex archwire–bracket interactions that occur.
Fabrication and characterization of nonmetallic archwires
McKamey and Kusy (1999) created a polymer–polymer composite wire, designed for use as a ligature, by encasing ultra‐high molecular weight polyethylene fibers in poly (n‐butyl methacrylate) formulated from a polysol containing benzoin ethyl ether. Subsequently, Zufall and Kusy (2000a, 2000b) created poly(chloro‐p‐xylylene)‐coated glass fiber–reinforced composite archwires made by photo‐pultrusion from S2‐glass and a polymer formed from bisphenol‐A diglycidyl methacrylate (Bis‐GMA) and triethylene glycol dimethacrylate (TEGDMA), with benzoin ethyl ether as an ultraviolet light photoinitiator. Strength and force decay were measured for the polymer–polymer composite archwires, and the frictional and viscoelastic behavior of the fiber‐reinforced polymer archwires was investigated extensively. Mathematical models were developed that described the in vitro behavior of the polymeric wires. Nonetheless, while laboratory results indicated that both polymeric wires would be suitable for their intended clinical applications, these wires were never introduced as commercial products, presumably because of the costs associated with developing the manufacturing techniques.
Besides the articles published by the Kusy research group that involved collaborations between biomedical engineers and orthodontists, other similar collaborative research on nonmetallic archwires has also been reported. Based on collaboration between the orthodontic faculty at the Hokkaido University School of Dentistry and an engineering faculty member at the Chiba Institute of Technology in Japan, Imai et al. (1998) reported a study of fiber‐reinforced polymeric archwires containing CaO–P2O5–SiO2–Al2O3 glass fibers that were longitudinally oriented in a polymethyl methacrylate matrix using a hot‐drawing method. The glass fiber surfaces of these wires were treated by a silane coupling agent to improve bonding with the polymer matrix. Collaboration between engineering mechanics, bioengineering, and orthodontics faculty at Tongji University in China and the National University of Singapore resulted in an article by Huang et al. (2003) on a tube‐shrinkage technique that was used to fabricate longitudinally oriented glass fibers (E‐glass) in an epoxy resin matrix. These authors noted that this novel technique did not have the problem of fiber damage or the limitation of a straight cylindrical geometry associated with the pultrusion (drawing) technique that had been previously used to fabricate unidirectional fiber‐oriented composite archwires.
It is important to acknowledge the extensive past research by Goldberg and Burstone, which also represented collaboration between a bioengineer and an orthodontist, on the development of fiber composites for a variety of dental applications, including orthodontic archwires. The publications from their past work can be easily found on PubMed, and in 2011 an exciting publication (Burstone et al., 2011) on polyphenylene polymer archwires appeared in the American Journal of Orthodontics and Dentofacial Orthopedics.
Fundamental studies of metallic archwires
Two important laboratory techniques that have been employed to investigate the mechanical properties and structures of nickel‐titanium orthodontic archwires, where these relationships have enormous clinical importance (Brantley, 2001), are differential scanning calorimetry (DSC; Lee et al., 1988; Khier et al., 1991) and dynamic mechanical analysis (DMA; Kusy and Wilson, 1990; Kusy and Whitley, 2007). Schematic illustrations of the experimental set‐ups for conventional DSC and DMA, along with schematic plots of the results from both techniques, are provided in Figures 22.4 and 22.5.

Figure 22.4 (a) Schematic experimental set‐up for conventional differential scanning calorimetry (DSC). (b) Schematic DSC plot for nickel–titanium orthodontic wire, showing enthalpy changes for heating (ΔHH) and cooling (ΔHC), start (As) and finish (AF) temperatures for transformation from martensite to austenite during heating, and start (MS) and finish (MF) temperatures for transformation from austenite to martensite on cooling.
Reproduced with permission from Kusy and Whitley (2007) / Elsevier.

Figure 22.5 (a) Schematic experimental set‐up for dynamic mechanical analysis (DMA). (b) Schematic DMA plot of changes in storage modulus for nickel‐titanium orthodontic wire, showing effects of transformation from martensite to austenite for heating and transformation from austenite to martensite for cooling, start and finish temperatures for both transformations, and temperature difference (hysteresis) between heating and cooling curves in transformation temperature range.
Reproduced with permission from Kusy and Whitley (2007) / Elsevier.
Conventional DSC provides information about temperatures for transformations between higher‐temperature austenite and lower‐temperature martensite phases, along with enthalpy changes (ΔH), and clearly differentiates among wires having true shape memory in vivo and the superelastic and nonsuperelastic wires (Khier et al., 1991). Temperature‐modulated DSC (Brantley et al., 2003), where a small sinusoidal thermal oscillation is superimposed on the linear heating/cooling ramp of conventional DSC, provides additional insight into transformations of nickel‐titanium wires (Figure 22.6), including occurrence of the intermediate R‐phase and a low‐temperature transformation within martensite, which was found to be twinning by transmission electron microscopy (Brantley et al., 2008).
With dynamic mechanical analysis, test specimens are subjected to an oscillating stress. Values are obtained for (i) storage modulus corresponding to the strain component in phase with stress (analogous to Young’s modulus for tension or shear modulus for that loading mode); (ii) loss modulus for the strain component 90° out of phase with stress; and (iii) tangent delta (ratio of loss modulus and storage modulus), which indicates the level of viscoelastic (nonlinear elastic) behavior. While the dynamic mechanical properties of stainless steel and beta‐titanium archwires did not vary over the DMA temperature range from −30 °C to 80 °C, differences in transformations observed by Kusy and Whitley (2007) for nonsuperelastic and superelastic archwires could have clinical significance. Values of transformation temperatures determined by DSC and DMA differ because the test specimens for the former technique are not subjected to stress during analysis, in contrast to the sinusoidal stress for DMA.

Figure 22.6 Temperature‐modulated DSC plots for (a) heating and (b) cooling of 35 °C Copper Ni‐Ti. Transformations involving austenite (A), martensite (M), and R‐phase (R) are designated by arrows. The R‐phase transformation in many orthodontic wires is not detected by conventional DSC analysis.
Reproduced with permission from Brantley et al. (2003) / Elsevier.
The mechanical properties of stiffness (elastic modulus), strength (force or stress for elastic limit, or maximum moment for elastic deformation), and range (extent of elastic deformation) are fundamental for the clinical performance of archwires. The relationship between these elastic properties and their measurement (which can be performed in tension, bending, or torsion) are discussed by Brantley et al. (2001), where previous research on measuring these properties by the Kusy group is also presented.
Rucker and Kusy (2002b) compared stiffness, strength, and range for three‐strand and six‐strand stainless steel wires with single‐strand stainless steel and nickel‐titanium leveling wires, as well as measuring the properties of wire segments in tension. They concluded that fabrication of multistrand stainless steel wires using alloys with enhanced tensile yield strength would allow these wires to compete better against conventional nickel‐titanium wires. Rucker and Kusy (2002c) used bending and tension tests to investigate the properties of noncoated single‐strand stainless steel and superelastic nickel‐titanium wires, nylon‐coated single‐strand and multistrand stainless steel wires, and noncoated multistrand stainless steel and nickel‐titanium wires. An important conclusion of this research was that few superelastic nickel‐titanium wires are activated sufficiently in vivo to exhibit superelastic behavior; that is, the superelastic plateau for unloading (Brantley, 2001).
In another important study, Kusy et al. (2004) investigated the composition, morphology, surface roughness, and sliding mechanics of six titanium‐based archwires using an SEM with x‐ray energy‐dispersive analyses, laser specular reflectance, and a friction testing machine. Five wires were beta‐titanium alloys with compositions similar to the original TMA (Ormco, Brea, CA, USA) product, and the sixth was an alpha–beta alloy containing 6 wt. % Al and 3 wt. % V. Beta‐titanium alloys are of particular clinical interest because of their biocompatibility due to the absence of nickel (Brantley, 2001). A variety of surface morphologies were observed, and specular reflectance and optical roughness measurements divided these wires into two groups. For values of angulation covering passive and active regions of sliding, coefficients of friction varied narrowly and were independent of surface roughness. Consequently, the authors suggested that from a clinical viewpoint, all six titanium‐based archwire products were comparable.
A study by Walker et al. (2007), involving collaboration between an orthodontist, a dental biomaterials faculty member, and an engineering faculty member, employed the three‐point bending test methodology in ANSI/ADA Specification No. 32. The elastic properties of 3M Unitek Beta III titanium and stainless steel archwires in distilled water, a neutral fluoride solution, and an acidulated phosphate fluoride solution were compared at 37 °C. The two fluoride solutions caused decreases in the clinically relevant unloading mechanical properties of both the beta‐titanium and stainless steel archwires, as well as changes in surface topography from corrosive attack. The important result from this study was that use of these fluoride solutions by patients could extend the time for orthodontic treatment.
Other studies on metallic archwires with a biomedical engineering focus reported relationships between surface condition and electrochemical behavior of nickel‐titanium wires and the use of diamond‐like coatings. Clarke et al. (2006) prepared a series of wires with a variety of surface conditions. Wires with very thick oxides contained high nickel contents in the oxide layer, and untreated samples with thicker oxides showed lower pitting potential values and greater nickel release in both long‐ and short‐term experiments. For long‐term immersion tests in 0.9% NaCl solutions, breakdown potentials for the oxide layers increased. Results demonstrated that appropriate surface treatment of nickel‐titanium wires is essential for optimum biomedical performance. Kobayashi et al. (2007) used an arc‐discharge ion‐plating process and deposited diamond‐like coatings (DLC) on nickel‐titanium orthodontic archwires. The wires were immersed in physiological saline at 37 °C for six months, and the concentration of nickel ions released from DLC‐coated wires was one‐sixth that from noncoated wires. The growth rate of squamous carcinoma cells on DLC‐coated wires was higher than that on noncoated wires. Wang and Zheng (2008) compared vacuum arc‐melted, hot‐rolled, quenched, and annealed plate specimens of a nickel‐titanium alloy containing 2.8% cobalt and the equiatomic nickel‐titanium alloy in deaerated artificial saliva. Potentiodynamic and potentiostatic test results showed that the corrosion behavior of the two alloys was similar, and that with increasing electrolyte pH, both the corrosion potential and the breakdown potential for pitting decreased. X‐ray photoelectron spectroscopy (XPS) revealed that the outermost passive film for the nickel‐titanium–cobalt alloy consisted mainly of TiO2, as found for nickel‐titanium. Inductively coupled plasma/optical emission spectroscopy (ICP/OES) indicated that nickel ion release from nickel‐titanium–cobalt was very close to that from nickel‐titanium, and neither titanium nor cobalt ions could be detected. This study emphasized the important in vitro relationship between corrosion behavior and pH underpinning the clinical use of nickel‐titanium orthodontic wires.
Force measurements
With the recent improvements in technology, there has been much interest in orthodontic force measurements. Friedrich et al. (1999) described three‐dimensional (3D) recording of orthodontic forces during treatment in vivo with fixed appliances, using brackets with a special design. Measurements were obtained by a six‐axis force‐torque sensor with strain gauge elements. Badawi et al. (2009) reported an in vitro method for 3D measurements and analyses of orthodontic force systems. Their orthodontic simulator was based on a single dental arch containing 14 teeth, to which brackets could be bonded and wires ligated. Using transducers, it was possible to measure simultaneously the force systems applied on all teeth in the arch by orthodontic appliances and generate 3D force displays. This article raised several controversies, with comments subsequently appearing in the Readers’ Forum of the American Journal of Orthodontics and Dentofacial Orthopedics (January and April 2010). It is evident that further research is needed to evaluate the biomechanics of conventional and self‐ligating brackets and their clinical efficacy.
Other investigations have examined the behavior of polymeric force‐producing modules. Stevenson and Kusy (1994) evaluated the effects of pre‐stressing, environmental acidity, oxygen content, and temperature on polyurethane chains. Specimens were treated for 10 and 100 days, and mechanical properties were compared with untreated specimens using stress‐relaxation tests. Conditioning treatments affected the residual load after relaxation, with the largest effect due to pre‐stressing. An increase in temperature significantly affected the deterioration of mechanical properties, while the acidity and oxygen content of the environment had no significant effects. In vitro force decay was described by a Maxwell‐Weichert model that assumed one mechanism was responsible for the rapid initial force loss and another mechanism was responsible for the slow force loss at longer times. While this model would ideally allow the orthodontist to predict the force supplied by these polyurethane chains at initial activation and any subsequent time during treatment, the in vivo environment to which the chains are exposed during clinical use causes complex degradation processes (Eliades et al., 2001a). The force decay of 3/16 in. latex elastics (3M Unitek, Monrovia, CA, USA) was evaluated by Wang et al. (2007). All specimen groups showed rapid force loss during the first hour, which had been reported previously. At later times of 24 and 48 hours, there was a highly significant force decrease in vivo and in artificial saliva, but no significant differences in a dry room. There was significantly more degradation for intermaxillary traction (where different times for wearing the elastics were important) than for intramaxillary traction, and for in vivo conditions compared with a dry room environment.
An earlier force measurement study was reported by Katona and Chen (1994), who performed an engineering analysis of a popular tensile bond strength testing methodology at that time for brackets bonded to enamel. They proposed that use of a long harness (wire loop) around the bracket constructed from thin ligature wire, pre‐stressing the harness, and lubrication may reduce the effects of inevitable load–bracket misalignment. These authors and their colleagues subsequently conducted numerous other studies on orthodontic force measurements and biomechanics, involving collaborations between orthodontics, biomechanics, and engineering faculty, and the corresponding publications can found on PubMed.
Orthodontic appliances and finite element analysis
Finite element analysis has been extensively employed in orthodontic research for three decades, with over 340 articles in PubMed. This technique, which has been utilized extensively by Katona, Chen and their colleagues, is a common procedure for solving biomedical engineering problems and is particularly useful for orthodontic biomechanics. Publications by this group (Viecilli et al., 2008, 2009; Meyer et al., 2010) illustrate the methodology and orthodontic applications.
Finite element analysis is a computational process that requires special software. A computer model of the system is initially subdivided into meshes. Using knowledge of elastic constants (Young’s modulus and Poisson’s ratio), computerized loading of the model causes deformation of the meshes, with displacements of their intersection points (nodes) that are converted to 3D strains and stresses using special finite element analysis software. Nonlinear elastic behavior can also be incorporated into finite element analyses.
An example of a model ceramic bracket with a network of meshes in shown in Figure 22.7. In an important earlier study, Ghosh et al. (1995) applied finite element analysis to several commercial ceramic brackets, observed the resulting stress distributions, and emphasized the value of this technique for investigating bracket design.

Figure 22.7 Example of bracket model with mesh pattern for finite element analyses of ceramic brackets.
Reproduced with permission from Ghosh et al. (1995) / Elsevier.

Figure 22.8 (a) Schematic experimental set‐up used to investigate stress–strain behavior of periodontal ligament. Schematic illustrations of clamped specimen for loading in extrusion (b) before and (c) after application of load.
Reproduced with permission from Toms et al. (2002) / Elsevier.
Several miscellaneous articles on orthodontic appliances, involving finite element analysis and other sophisticated approaches in collaborations between biomedical engineers and orthodontists, were also retrieved using PubMed. Lewis et al. (1996) used finite element analysis to determine stresses in the adhesive layer of two‐dimensional models of bonded brackets. Liu et al. (2004b) used strain tensor analysis to investigate effects of chin cup therapy on the mandible in Class III malocclusions. Lin et al. (2004) utilized finite element analyses to study the effect of retainer thickness on posterior resin‐bonded prostheses. Pilliar et al. (2006) developed a finite element model to investigate crestal bone loss patterns around sintered porous‐surface and machined‐threaded implants produced for orthodontic anchorage that were used in an animal study. Qi et al. (2007) measured the transmission of forces for a cast‐metal, fixed, twin‐block appliance used to achieve rapid correction of Class II malocclusions. The very wide scope of these articles illustrates the range of research that can be performed in this area and should encourage future collaboration between biomedical engineers and orthodontists.
Miscellaneous areas of research
In the first of three diverse articles, Liu et al. (2004a) investigated the genotypic stability of Streptococcus mutans using plaque samples obtained from supragingival smooth surfaces of right maxillary teeth at four stages of orthodontic treatment. DNA was prepared from a large number of S. mutans strains, and strains were identified using PCR. PCR fingerprinting was applied to determine genotypes. The amount of S. mutans increased significantly after fixed appliances were bonded, and S. mutans clones were stable during orthodontic treatment.
In the second article, Kusy (2004) presented a theoretical bioengineering model that considered the influences of specific concentrations of allergens on their clinical effects, focusing on nickel and chromium for orthodontic patients. He noted clinical responses to these two allergens in a large number of patients. The practical application of his model involved a questionnaire, and in the patient reporting an allergy a skin patch test would be administered to establish whether alternative alloys not containing these elements should be used during treatment.
The third article was by Toms et al. (2002), who investigated the stress–strain behavior of the periodontal ligament (PDL) using tissues obtained from cadaveric specimens of young and elderly adults. The schematic experimental set‐up is shown in Figure 22.8, and a representative stress–strain curve for the PDL is presented in Figure 22.9. The stress–strain curves for both intrusion and extrusion loading had nonlinear behavior with distinct low elastic modulus toe and higher elastic modulus linear regions. The loading behavior of the PDL was found to be dependent on age, location (cervical margin, apex region, and midroot region), and load direction (intrusion or extrusion). An important consequence from this study was the suggestion that computer simulations of orthodontic tooth movement should incorporate the nonlinear behavior of the PDL.

Figure 22.9 Nonlinear stress–strain curve for the periodontal ligament at low stress. Toe modulus was calculated for toe region, beginning at origin, and linear modulus was calculated in linear region shown. Modulus values were calculated for intrusion and extrusion.
Reproduced with permission from Toms et al. (2002) / Elsevier.
Current research activities and potential future applications
Advances in materials science and related fields have resulted in the introduction of materials and processes that create applications of unique properties in a wide range of biomedical disciplines. The purpose of this part of the chapter is to highlight advances (Box 22.2) that may have direct application to orthodontic materials from both the clinical and research perspectives. The majority of these advances have not yet been applied to orthodontic materials; in some areas preliminary data on experimental products have been published, although without current translation into commercial availability.

VIDEdental - Online dental courses
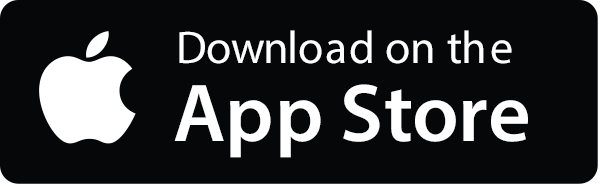
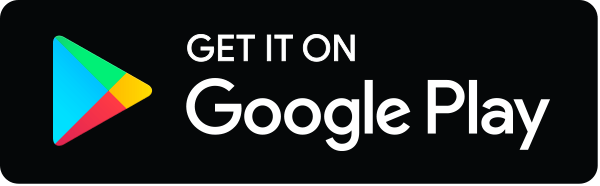