The metabolism of proteins and amino acids
Publisher Summary
This chapter discusses the metabolism of proteins and amino acids. Proteins play an essential part in every biological activity, and as they are built up from about twenty different amino acids, their metabolism, like their structure, is complex. Normal adults of any mammalian species are in a state of nitrogen balance in which the total amount of nitrogen lost from the body, most of which is derived from protein, exactly balances the amount of nitrogen that is consumed in the diet. During growth, pregnancy, and recovery from injury or wasting disease, the nitrogen intake is likely to exceed the nitrogen output as new tissue is being formed. When there is retention of nitrogen in this way, the subject is said to be in positive nitrogen balance. Negative balance and a net loss of nitrogen from the body occur when the protein intake is reduced during a wasting disease and almost imperceptibly in ageing. Protein molecules are too large to be transported through the gut wall to the bloodstream by the normal absorptive processes. Even if this were possible, it would be dangerous because foreign proteins cause toxic reactions in the body. The first step in the metabolism of food proteins is their hydrolysis to amino acids by the proteolytic enzymes of the gastrointestinal tract. Amino acids are rapidly absorbed in the intestine. The intestinal wall is lined with specialized absorptive cells whose primary function is the transport of nutrients from the lumen of the gut into the portal circulation. These cells contain active transport systems for both sugars and amino acids in the brush border membrane.
Proteins play an essential part in every biological activity, and since they are built up from about twenty different amino acids, their metabolism, like their structure, is complex. The present chapter is planned to give only a general picture of protein and amino acid metabolism. Examination of the individual metabolic pathways of the various amino acids is beyond the scope of this book. Protein biosynthesis, which is dependent on the nucleic acids, is dealt with in Chapter 20.
Protein digestion
As the food proteins pass along the gastrointestinal tract they undergo a systematic attack, being first subjected to three endopeptidases (pepsin, trypsin and chymotrypsin) which act on proteins and large polypeptides, splitting them at definite points along the chain. The peptides so produced are then subjected to the action of several types of exopeptidase which break off terminal amino acids. A list of the proteolytic enzymes of the gastrointestinal tract is given in Table 19.1.
Table 19.1
The main proteolytic enzymes of the digestive tract
Enzyme | Source | Mode of activation | Linkages preferentially attacked | Optimum pH | |
Zymogen form | Active form | ||||
Endopeptidases | |||||
Pepsinogen | Pepsin | Gastric mucosa | Hydrochloric acid and autocatalysis | Those in which Phe, Tyr or Trp contribute the −NH−group | 1·5−2·0 |
Trypsinogen | Trypsin | Pancreas | Enterokinase and autocatalysis | Those in which Lys or Arg provide the −CO−group | 7·0−9·0 |
Chymotrypsinogen | Chymo-trypsin | Pancreas | Trypsin | Those in which Phe, Tyr, Trp and, to a lesser extent, Leu and Met contribute the −CO− group | 7·5−9·0 |
Exopeptidases Procarboxypeptidase | Carboxypeptidase | Pancreas | Trypsin | The bond linking the C-terminal amino acid to the rest of the chain | 7·2 |
– | Amino-peptidase | Intestinal epithelium | – | The bond linking the N-terminal amino acid to the rest of the chain | 7·4 |
– | Dipeptidase | Intestinal epithelium | – | The bond joining two amino acids to form a dipeptide |
Amino acid absorption
Thus the energy for the active transport of amino acids is derived indirectly from the hydrolysis of ATP (Figure 19.1). After uptake into the absorptive cells by this method, the amino acids pass into the portal circulation by a passive transport process. The brush border membrane contains a number of different transport systems for amino acids, which have overlapping specificity. It is probable that similar systems are responsible for the uptake of amino acids into other tissues such as kidney and liver. As a result of the operation of such transport systems, the total free amino acid concentration in the plasma is kept at between 2 and 4 mM but in the tissues it is between 15 and 30 mM. Amino acids derived exogenously from the food are mixed with amino acids derived endogenously from the tissues to form a metabolic pool. The essential amino acids (see below) are taken up by the tissues with great avidity and the non-essential amino acids, notably glycine, alanine, glutamic acid and glutamine, account for 80% of the total free amino acid nitrogen.
Essential amino acids
Although plants and many microorganisms are able to synthesize all the amino acids they require from simple carbon compounds and non-specific sources of nitrogen such as ammonia, higher animals are unable to do this and must obtain some of the amino acids from the protein in the diet. An essential amino acid is one that an animal is either unable to synthesize for itself or which it cannot synthesize at a sufficient rate to meet the needs for metabolism and growth. Different species vary to some extent in their essential amino acids. Humans require at least eight and probably ten. They are valine, leucine, isoleucine, lysine, methionine, phenylalanine, threonine and tryptophan with arginine and histidine having a doubtful status.* The latter are ‘relatively indispensable’ in that they can be synthesized in the body but their rate of synthesis may be too slow fully to supply the needs of the growing child. On a similar basis glycine is an essential amino acid for rapid growth of feathers in young birds such as the chick. Young rats require the same ten amino acids as are needed by children.
The quantities of the various essential amino acids required daily in the diets of individual humans were determined by Rose who fed young adult volunteers on diets containing adequate amounts of purified carbohydrate, fats, minerals and vitamins but provided mixtures of amino acids in the place of protein. The mixtures contained all the amino acids except the one under investigation which was given separately and, by slight alterations in the level of intake, the subject could be made to go reproducibly from positive to negative balance and back again. This indicated the daily requirement of the individual for this amino acid. The experiment was repeated for each essential amino acid in turn thus giving a picture of the overall needs of that person. Particular individuals were found to have well-defined requirements although there was considerable variation from one person to another. Average results for men, women and infants are given in Table 19.2. As mentioned in Chapter 10 the ‘safe’ level of a given amino acid is taken as being considerably higher than the minimum requirement.
Table 19.2
Estimated daily requirements for the essential amino acids
Amino acid | Minimum requirement | ||
Infants(mg kg−1) | Men(g) | Women(g) | |
Arginine | * | 0 | 0 |
Histidine | 34 | 0 | 0 |
Isoleucine | 126 | 0·7 | 0·55 |
Leucine | 150 | 1·1 | 0·73 |
Lysine | 103 | 0·8 | 0·54 |
Methionine (in the presence of cysteine) | 45 | 1·1 | 0·70 |
Phenylalanine (in the presence of tyrosine) | 90 | 1·1 | 0·70 |
Threonine | 87 | 0·5 | 0·37 |
Tryptophan | 22 | 0·25 | 0·17 |
Valine | 105 | 0·8 | 0·62 |
Amino acid metabolism
A summary of amino acid metabolism is given in Figure 19.2. Amino acids are used for protein synthesis and as N and C donors for the synthesis of other types of macromolecule, e.g. the nucleic acids as well as numerous small molecular compounds. After deamination, i.e. removal of the amino group, the carbon skeleton may be used for the formation of glucose or even fats or it may be oxidized to CO2 and water with the production of metabolic energy. Decarboxylation, i.e. removal of the carboxyl group of certain of the amino acids, leads to the production of biogenic amines such as histamine, serotonin and γ-aminobutyrate.
Tissue interrelationships in amino acid metabolism
The metabolism of amino acids proceeds by pathways which are common to most tissues, but the pathway for the conversion of ammonia to urea occurs only in the liver. During the degradation of amino acids in peripheral tissues such as skeletal muscle, the ammonia formed is not released directly into the bloodstream. Instead it is used to form the amino acids alanine and glutamine from pyruvate and glutamate which are readily available, and it is these amino acids that are then released. The alanine produced by the tissues is taken up by the liver and converted to urea and glucose. Although some glutamine is metabolized by the liver, the major site of glutamine metabolism is the intestine where it is used as a major respiratory fuel. The ammonia produced by glutamine metabolism in the gut returns immediately via the portal circulation to the liver, where it is detoxified. These tissue interrelationships in amino acid degradation are illustrated in Figure 19.3.
Pathways of amino acid catabolism
Transamination and deamination
< ?xml:namespace prefix = "mml" />
Transaminase reactions are freely reversible so that they function in both the synthesis and breakdown of amino acids. All transaminases require pyridoxal phosphate, a derivative of vitamin B6 (page 165), as a cofactor which transfers the α-amino group from an amino acid to a keto acid. In general, transaminases have a high Km value for the appropriate amino acid but a much lower Km for 2-oxoglutarate.
The fate of the carbon skeleton
In general, the deaminated residues of the various amino acids are converted into intermediates of the citrate cycle, acetyl-CoA or acetoacetyl-CoA. The pathways involved are long and complex and will not be considered in detail. An outline of the metabolic fate of the various amino acids is given in Figure 19.4. The amino acids which produce pyruvate, 2-oxoglutarate, succinyl-CoA, oxaloacetate or fumarate are said to be glucogenic
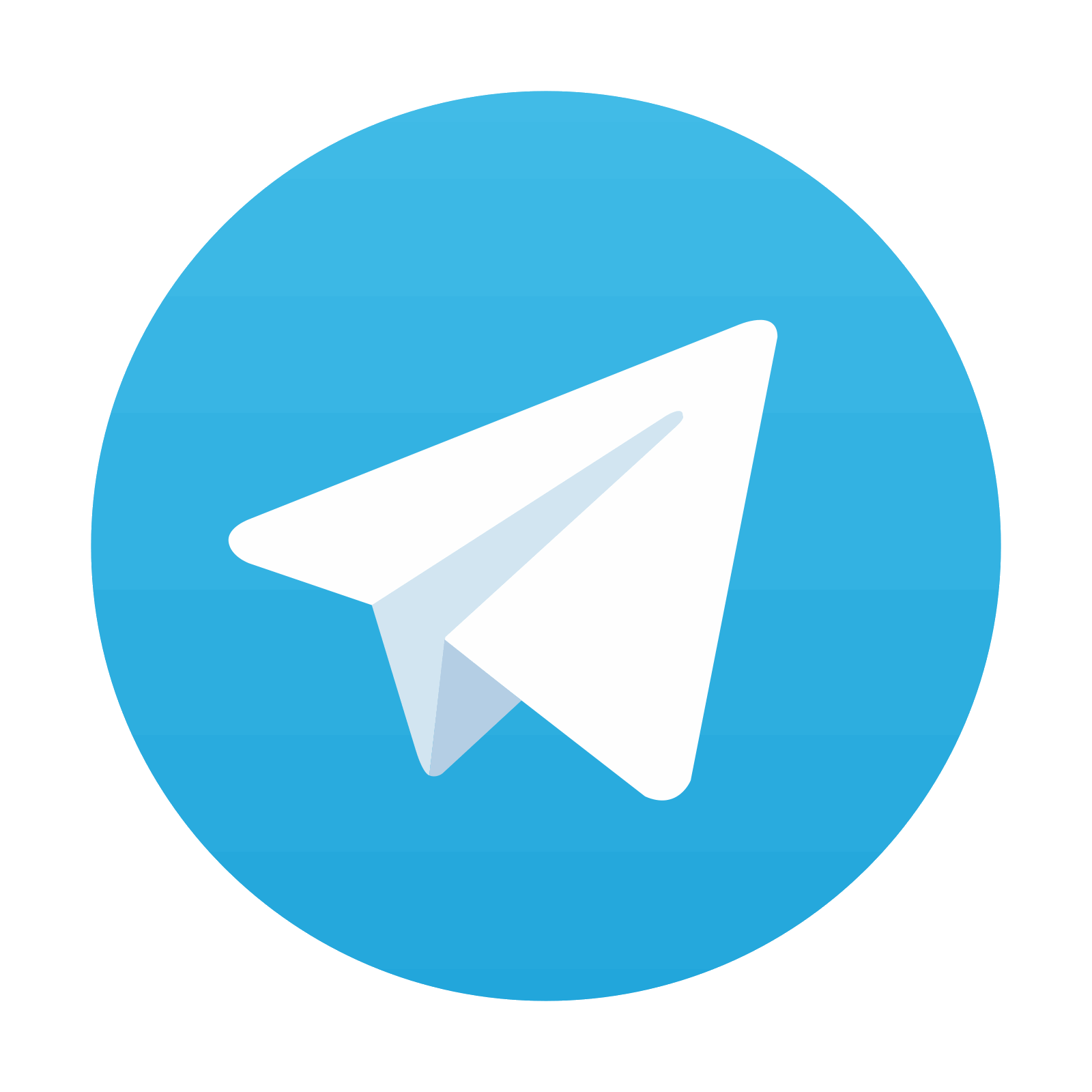
Stay updated, free dental videos. Join our Telegram channel

VIDEdental - Online dental courses
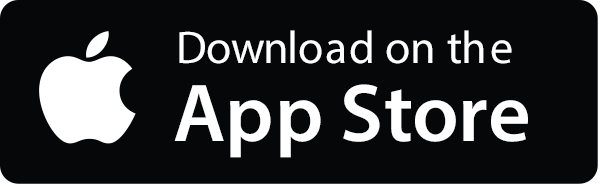
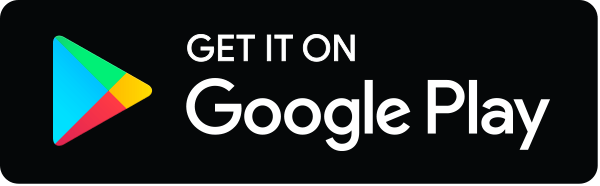