Highlights
- •
TPO and CQ-based experimental resin composites were prepared and their physical properties analyzed.
- •
The use of TPO with appropriate curing parameters leads to improved conversion.
- •
Mechanical properties in TPO-composites were improved compared to the CQ-controls.
- •
Polymerization stress levels were similar between TPO and CQ-resin composites in some cases.
- •
Temperature rise during polymerization measured in a tooth model were well below 5.5 °C.
Abstract
Objectives
to complement our previous work by testing the null hypotheses that with short curing times and high DC, TPO-based resin composites would exhibit (1) higher polymerization stresses and consequently display (2) higher temperature rise and (3) higher flexural modulus, flexural strength and hardness, compared to a conventional CQ-based experimental composite.
Methods
Two experimental resin composites using either Lucirin-TPO or camphorquinone/DMAEMA as photoinitiators were prepared. Light curing was carried out using spectral outputs adapted to the absorption properties of each initiator. Different irradiation protocols were selected (0.5, 1, 3, 9 s at 500, 1000 and 2000 mW/cm 2 for Lucirin-TPO based composites and 20 or 40 s at 1000 mW/cm2 for Lucirin-TPO and camphorquinone-based composites). Degree of conversion (DC) was measured in real time by means of FT-NIR spectroscopy. Pulpal temperature rise (Δ T ) was studied in a tooth model. Polymerization stress was monitored using the Bioman instrument. For cured specimens, flexural modulus and flexural strength were determined using a three point bending platform and Vickers hardness was determined with a microhardness indentor on samples prior to and after 24 h incubation in 75/25 ethanol/H 2 O. Premolars were restored with both materials and microleakage at the teeth/composite interfaces following restoration was assessed.
Results
Lucirin-TPO-based composites irradiated at radiant exposures of 3 J/cm 2 and more exhibited significantly higher DCs, associated with increased flexural moduli and hardness compared to CQ-based composites. For an ultra-short irradiation time of 1 s at 1000 mW/cm 2 , TPO-composites displayed similar polymerization stresses compared to CQ-controls with yet a 25% increase for flexural modulus and 40% increase for hardness measured after EtOH/H 2 O sorption. Higher stress rates were however observed in all curing protocols compared to CQ-composites. Microleakage was similar between TPO and CQ-composites irradiated at 1000 mW/cm 2 for 3 and 20 s respectively, while a significant increase was observed for TPO-composites irradiated for 1 s. Δ T measured through a 0.6 mm thick dentin layer were all below 5.5 °C; TPO-composites exhibited similar or lower values compared to controls.
Significance
The use of Lucirin-TPO in resin composites along with appropriate curing conditions may allow for a major reduction of irradiation time while improving mechanical properties. The amount of stress observed during polymerization in TPO-based composites can be similar to those using CQ and the cohesion at the restoration-tooth interface was not affected by short curing times. Contrary to other studies, we found that the temperatures increases measured during polymerization were all well below the 5.5 °C threshold for the pulp.
1
Introduction
According to clinical studies, the major reasons for failure of resin composite restorations are secondary caries, restoration fracture and need for endodontic treatment . From a biomimetic standpoint, the materials used should display the same characteristics as the replaced tissues. For dentin, hardness lies in the range of 50–60 HV , elastic modulus is around 20–25 GPa . Current commercial resin composites exhibit flexural moduli and strength in the range 2.4–14.7 GPa and 45–145 MPa, respectively and Vickers hardness falls in the range of 18.6–77.7 HV/20 . The wide variation of results may be explained by the different formulations but also the quality of conversion as higher degrees of conversion (DC) usually lead to more rigid and harder structures . Besides mechanical improvement, the materials should present a reliable interface with the dental tissues (generating minimal shrinkage stress on cure) and be safe for the pulp tissue (low monomer elution, low temperature rise in the pulp chamber, etc.). At the same time, resin composites should meet the patients and practitioners requirements, notably the reduction of chairside procedures times, for example by shortening curing time.
Despite the clear clinical interest of reducing curing time by improving polymerization kinetics, higher rates could result in greater stresses at the dentin-composite interface, which may in turn induce cusp deflection and/or gaps . However the development of polymerization shrinkage stresses is a complex phenomenon, which was shown to be affected by multiple factors including not only polymerization rate, but also degree of conversion, volumetric shrinkage and elastic modulus . For example, polymerization stress was shown to evolve non-linearly with conversion while volumetric changes are the combination of both contraction due to the polymerization and contraction/expansion related to thermal effects . Finally, an additional possible concern of faster polymerization with higher conversion and higher irradiances is the heat generated during polymerization, which could damage the underlying pulp . Besides, reaction temperature rise (Δ T ) was also positively correlated to polymerization shrinkage .
In a recent paper by our group, using a model experimental BisGMA/TegDMA composite formulation, we demonstrated the possibility of improving the degree of conversion (DC) and significantly reducing monomer elution despite a significant reduction of curing time (1 s for a 2 mm composite layer at 1000 mW/cm 2 ) . This was achieved first by replacing the common ketone (camphorquinone)/amine photoinitiation system (CQ) with a Norrish Type I monoacylphosphine oxide photoinitiator, namely Lucirin-TPO (TPO), and also by choosing appropriate irradiation parameters (wavelength range, irradiance and irradiation time). Several other studies have already demonstrated that the curing light source is critical in realizing the potential of TPO and that switching from CQ to TPO, and other photoinitiators with higher molar absorptivity is advantageous for materials development . Further, the clinicians’ desire for reduced curing time (3 s, or less) has seen an influx to the dental market of high irradiance (>2000 mW/cm 2 ) LED light curing units that may not provide sufficient cure of some composite material types . If such short curing times are desired, there is a requirement for the development of materials chemistry, rather than simply increasing the power of the curing light source. Overall, both intrinsic and extrinsic parameters should be taken into account in order to optimize the polymerization efficacy and resulting material properties .
Despite these clear improvements, it remains unclear whether the dramatic increase in reaction speed seen in TPO-based composites , associated with improved conversion and mechanical properties , will be detrimental in terms of shrinkage stress at short curing times. Since the generation of polymerization stress throughout cure is not an intrinsic material property, its comprehensive evaluation requires the investigation of other central parameters, i.e. degree of conversion, temperature rise and elastic modulus. Hence, the aim of the present study was to complement our previous work by testing the null hypotheses that with short curing times and high DC, TPO-based resin composites would exhibit (1) higher polymerization stresses and consequently display (2) higher temperature rise and (3) higher flexural modulus, flexural strength and hardness, compared to a conventional CQ-based experimental composite cured for 20 or 40 s at 1000 mW/cm 2 .
2
Materials and methods
2.1
Materials
Two experimental composites materials were prepared, based on a 70/30 wt% Bis-GMA/TegDMA resin (Sigma–Aldrich), using two different photoinitiators systems in equimolar concentration (1.34 × 10 −5 mol cm −3 ): either camphorquinone and dimethylaminoethyl methacrylate (altogether CQ, from Sigma Aldrich) or Lucirin-TPO (TPO, from BASF). Initiators accounted for 0.2/0.8 wt% and 0.42 wt% for CQ and TPO, respectively. Fillers were silanated barium glass (G018-186/K6, d 50 = 3 ± 1 μm, Schott AG, Landshut Germany) and methacrylsilane treated fumed silica (12 nm, Evonik Industries, Germany) introduced in amounts of 65/10 wt% respectively. The fillers were dispersed using a dual asymmetric centrifuge (Speed mixer, FlackTek, USA).
2.2
Flexural bending test
Rectangular specimens for the flexural bending test were polymerized in Teflon moulds of dimensions 25 × 2 × 2 mm ( n = 5 following ISO4049). Light-curing was carried out with an AURA light device (Lumencor, USA) providing narrow spectral outputs. Irradiation could be applied independently at two wavelength ranges: 395–415 nm and 455–485 nm for TPO and CQ-based composites, respectively ( Fig. 1 ). Light irradiance calibrations were carried out as described previously . Irradiation was applied in 5 successive and non-overlapping cycles starting at the center of the sample, then extended towards the ends. During each irradiation, Mylar films were used to cover the top and bottom surfaces of the samples and opaque masks with a 5 mm diameter window (the dimension of the light guide) were used to cover the adjacent sample areas and thereby reduce overlapping irradiation areas due to light diffusion. The bars were stored for 24 h in the dark at room temperature before being tested.
The CQ-based composites (controls) were irradiated for 20 and 40 s at 1000 mW/cm 2 . TPO-composites were irradiated at three different irradiances for: 2000 mW/cm 2 for 9, 3, 1 or 0.5 s, 1000 mW/cm 2 for 40 s 20, 9, 3, 1 or 0.5 s and 500 mW/cm 2 for 9, 3, 1 or 0.5 s.
Flexural testing was conducted using a universal testing machine (Lloyd LRX Plus, Lloyd Instruments), with a 20 mm span between supports and 0.75 mm/min crosshead speed. Flexural modulus was calculated according to Eq. (1) and flexural strength was calculated according to Eq. (2) , where F is the load (N), l is the distance between supports (mm), w and t are the width and thickness of the sample (mm) and d is the deflection due to the load F (mm).
2.3
Vickers hardness
5 mm diameter, 2 mm thickness disc-shaped specimens were prepared in split Teflon moulds and were irradiated following the same protocol as described in the previous section ( n = 3). Following irradiation, the Mylar films were removed, samples were extracted from the moulds and were stored for 24 h in the dark at room temperature before being tested. A first series of hardness measurements was then carried out. Subsequently, samples were incubated for another 24 h in 75/25 vol% ethanol/H 2 O; period after which hardness was again measured. In both cases, measurements were carried out on the upper surface (two per surface) with a microhardness tester (Durimet,Leitz, Wetzlar, Germany) using a 100 g load ( F = 0.1 kgf) and 30 s indentation period. Hardness calculation was done using mean diagonal values of indentations ( d ) and Eq. (3) .
2.4
Polymerization stress
The stress generated during the polymerization reaction was measured using a single cantilever device, the Bioman instrument, as previously described . In the present study the specimen layer was restricted to 0.9 ± 0.05 mm to limit the flowing of the material. The light source was located below the glass slide at a distance of 7.5 mm from the bottom surface of the sample and calibrations were repeated in order to obtain actual irradiances of 500, 1000 and 2000 mW/cm 2 . The force (stress) generated by the material was measured via the strain-gage signal of the cantilever load cell and using Eq. (4) ( n = 3).
σ p = k Δ V S
where Δ V is the voltage difference read at the sensor during the measurement, k is a constant such as k Δ V is equivalent to Newtons and S is the surface of the sensor (mm 2 ).
Maximum polymerization stress was determined as the value reached after 4 min of acquisition and rates were calculated as the first derivative against time.
2.5
Temperature analysis
The temperature variations associated with polymerization and irradiation were monitored using an experimental setup. A composite specimen was placed in a Teflon mold (2 mm thick, 5 mm inner diameter), itself resting on a molar tooth which was machined to leave a 0.6 mm thick dentin roof, accommodate a temperature sensor in the pulp chamber and present a flat occlusal surface ( Fig. 2 (a) ). The tooth was partially immersed in a temperature-controlled water bath (37 ± 1 °C) as shown in Fig. 2 b). Irradiation was carried out as in other analyses, with the light guide in contact with the Mylar strip covering the composite specimen. Temperature was recorded with a 4-wire platinum sensor and data acquisition module (HSRTD-3-100-A-1 M and PT-104A respectively, Omega, UK) every 0.1 s with an accuracy of 0.15 °C ( n = 3). The maximum temperature changes are reported (Δ T ).
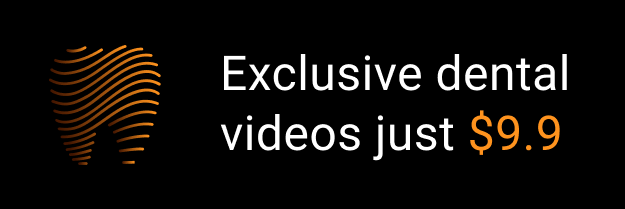