Abstract
Objectives
Secondary caries limits the longevity of restorations and is thought to be associated with faulty restorations, e.g. dentin-restoration interfacial gaps. Recent evidence indicates that loading of restorations might aggravate the effects of gaps on interfacial mineral loss. It is unclear if this effect of loading is dose-dependent or not, and if restoration material properties like elasticity moderate the association between load and mineral loss. We hypothesized that mineral loss of secondary lesions increases with increasing load, and that this association is moderated by the elastic modulus of the placed restoration material.
Methods
Dentin-restoration specimens with simulated interfacial gaps were submitted to cariogenic Lactobacillus-rhamnosus-biofilms for 10 days, and concurrently loaded with different loads (0/42/84/126 g per specimen, n = 12/group). Two different composites (LEC: low elastic-modulus composite, HEC: high elastic-modulus composite) were employed. Transversal microradiography was used to evaluate the superficial and interfacial (wall) lesion mineral loss. Generalized linear modeling (GLM) was used to evaluate the association between loading, material and their interaction on mineral loss.
Results
Surface mineral loss was not significantly associated with loading, material, or their interaction (p-values ranged between p = 0.062 and 0.526). For deep interfacial (wall) lesions, the applied load (p = 0.023) but not the material (p = 0.382) showed a significant effect. The interaction between both significantly affected mineral loss (p = 0.01). Loads of ≥84 g per specimens were associated with higher wall lesion mineral loss.
Conclusions
Loads above a certain threshold significantly increased interfacial (wall) lesion mineral loss. This association was moderated by the elasticity of the placed restoration materials.
Clinical significance
The clinical relevance of our findings remains unclear, as future studies are needed to understand how exactly both load and material elasticity affect secondary lesion induction.
1
Introduction
Secondary caries is stated to be the main reason for long-term failure of dental composite restorations, especially in high caries risk patients , limiting the restoration lifespan, generating high costs for replacement, and eventually shortening the time restored teeth are retained . There is great ambiguity as to the nature of secondary caries: For example, is it claimed that secondary carious lesions are merely primary carious lesions adjacent to restorations, without any or very limited association with the adjacent restoration . However, secondary lesions might be associated with the adjacent restoration: The surface roughness of a material, but also a possible antibacterial effect might affect biofilm adhesion and retention in the area around the tooth-restoration interface; remineralizing or buffering materials might moderate possible acid-related demineralization of tooth tissues .
In addition, faulty restorations, especially those with interfacial gaps, seem to increase the susceptibility for secondary lesions, which consequently consist of a surface (outer) and a wall (interfacial) lesion as a result of fluid (acid) exchange or biofilm penetration into the faulty interface . A number of studies found the gap size itself to be an important factor associated with interfacial mineral loss . In small gaps, the diffusion of acids in and dissolution products out of the gap might limit the speed of lesion induction, while larger gaps might not limit the diffusion and thus lead to greater mineral losses of the wall lesions .
For small gaps, the fluid and biofilm exchanges seem to be moderated by masticatory forces, as has been shown both in vitro and in clinical studies . So far, there is limited evidence as to nature of the association between loading and mineral loss: First, one in vitro study showed that greater loading also increased the lactic-acid induced mineral loss , but it remaind unclear if this also applies to biofilm-induced mineral loss (as would occur clinically). Second, it is unknown if any dose-effect interaction follows a linear or other continuous association, or is characterized by a threshold effect, with certain minimum loads being required to facilitate fluid exchange or biofilm penetration along the interface. Last, it has not been assessed if material properties moderate the effects of loading: Different material elasticity might, in theory, lead to different translation of the load onto the interfacial fluid, with dampening or pumping effects reducing or increasing the effects of load.
The present study investigated how different loading of different composite restorations impacts on the development of secondary carious lesions. We hypothesized that mineral loss of secondary lesions increases with increasing loads, and that this association is moderated by the elasticity of composite material.
2
Methodology
2.1
Study design
In the present study, miniature dentin-restoration specimens with simulated interfacial gaps were submitted to a cariogenic biofilm to induce secondary caries lesions. Concurrently, specimens were loaded occlusally, with different loads being applied. To test if the used restorative composite material moderated the association between loading and mineral loss, two different composites were employed: LEC- Low elastic modulus composite (Tetric Evo Flow, E-Modulus 5100 MPa, Ivoclar Vivadent, Schaan, Liechtenstein) and HEC- High elastic modulus composite (Tetric Evo Ceram, E-Modulus 10000 MPa, Ivoclar Vivadent, Schaan, Liechtenstein). While the elastic modulus of these composites was hypothesized to be the variable of interest for moderation of loading effects, we further characterized both materials for surface roughness on polished and non-polished surfaces, bacterial adhesion susceptibility, and buffering capacity (see Appendix A ). While both polished and non-polished surface roughness was lower for LEC than HEC, neither the bacterial adhesion susceptibility nor the buffering capacity was found to differ significantly between composites (see Appendix A ).
2.2
Specimens preparation
From 96 bovine incisors, 96 dentin slabs (2 × 3 × 6 mm) ( Fig. 1 a) were prepared (Band Saw 300cl, Exakt Apparatebau, Norderstedt, Germany) and polished (silicon carbide papers 1200, 2500, 4000 grit; Struers, Willich, Germany). Dentin slabs were embedded in methyl methacrylate resin (Technovit 4071, Heraeus Kulzer, Hanau, Germany) leaving the top and one side unprotected ( Fig. 1 b). Afterwards, specimens were randomly allocated to the two materials, LEC and HEC. On the top surface of the dentin specimens a self-etch adhesive (Scotchbond Universal, 3 M ESPE, St. Paul, USA) was applied leaving an unbonded U-shaped window (2 × 2 mm) ( Fig. 1 b). In this window, a 2 mm wide and 100 μm thick copper foil (Gemmel Metalle, Berlin, Germany) was placed, the first composite increment build up around the margin of the copper foil to fix it in place, and light-cured for 20 s (Valo, Ultradent, Salt Lake City, USA) at 1400 mW/cm 2 )( Fig. 1 c). A 2 mm thick composite layer was applied in bulk on the top surface of the dentin specimens and light-cured as described for 20 s ( Fig. 1 d). The copper foil was carefully removed, leaving a mean (SD) gap of 145 (25) μm between the composite and the dentin specimen ( Fig. 1 e). Gap size was not significantly different between any of the groups (p ≥ 0.05/t-test). The composite and dentin surfaces were finally polished with water-proof silicon carbide papers (4000 grit) and the patency of the gap was checked again using the copper foil.

Specimens were fixed to the holding plate using self-cure resin and the whole assembly (distribution disc and holding plate with the specimens) was sterilized (121 °C, 2.1 bar, Tuttnauer 3870 ELV, Biomedis, Gießen, Germany) and ready to be attached in the simulation chamber.
2.3
Bacterial culture
Lactobacillus rhamnosus GG (LGG, DSM 20021, DSZM, Braunschweig, Germany) was cultured for 48 h (37 °C, 5% CO 2 ) on de-Man-Rogosa-Sharpe agar (MRS, Difco, Franklin Lakes, USA). Cultures were inoculated into 2.5 l MRS medium with 1% sucrose (MRS-S) and grown for 24 h.
2.4
Masticatory biofilm model
To simulate oral conditions, a custom-made masticatory biofilm model which allowed simulating both mechanical and biological challenges concurrently was used. The model consisted of four load distribution discs ( Fig. 1 f), with miniature specimens being loaded via adjustable stainless steel pins (0.5 mm diameter). The discs were mounted in an artificial mouth chamber within a dual-axis chewing simulation machine (Kausimulator CS-4.8, Willytech, Feldkirchen-Westerham, Germany). Per discs, 24 specimens were loaded.
Specimens were randomly allocated to one of the four load distribution discs. Each of these discs was loaded differently, with loads 0, 1, 2, or 3 kg per disc, equaling effective loads of 0, 42, 84 or 126 g per specimen (total weight/number of specimens), respectively (n = 12/group). Loading was applied centrally onto the interfacial gap ( Fig. 1 e) with a frequency of 0.16 Hz, 4 s loading and 2 s of unloading. All specimens were subjected to a total of 144,000 loading cycles (equaling 10 days of chewing simulation at a loading frequency of 10 strokes per min).
After mounting the discs within the artificial mouth chamber, an LGG biofilm was cultured at 100% humidity and 37 °C. Fluid flow within the chamber was realized by computer-controlled peristaltic pumps (Seko PR1, Rieti, Italy). Three times daily (every eight hours), a simulated meal was provided via 1 l MRS-S solution for 30 min, followed by an immersion time of 30 min, removal of the fluid, a simulated salivary flow using 1 l sterile Modified Defined Mucin Medium (DMM) medium for 30 min, a saliva immersion time of 30 min, and removal of saliva. In the first of these daily cycles, we additionally provided LGG (approximately 7 × 10 6 CFU/ml) overnight culture medium.
2.5
Transversal microradiography (TMR)
After a 10 days simulation period, specimens were removed and cut (Band Saw 300cl) perpendicular to the surface and the interfacial gap for mineral loss analysis. The cut surface was attached to an object holder and ground (silicon carbide papers 1200, 2500, 4000 grit) to a thickness of 100 ± 10 μm (Exact 400 CS; Exact). The thin sections were exposed to radiation (PW2213/20 tube, Panalytical, Kassel, Germany; PW 1730/10 generator, Philips, Eindhoven, The Netherlands) at 20 kV and 10 mA with an exposure time of 10 s. The exposed films (Fine 71337, Fujifilm, Tokyo, Japan) were developed according to manufacturer’s instructions under standardized conditions. The obtained microradiographs were digitally analyzed using a transmitted light microscope and a CCD-video camera (XC 77 CE, Sony, Tokyo, Japan). Mineral loss ΔZ (vol% × μm) was measured using TMR for windows version 2.0.27.2 (Inspector research, Amsterdam, The Netherlands). Mineral loss was measured at four different areas: twice along the interfacial gap to determine the wall lesion extend (WM 1 at 400 μm distance from the outer surface, WM 2 at 800 μm distance from the outer surface) and twice along the outer surface to determine surface lesions (SM 1 at 400 μm distance from the interfacial gap, SM 2 at 800 μm distance from the interfacial gap) (Figs. 1 e, 2 a, b). Some specimens were lost during preparation, the number of specimens is thus given in the results part.

2.6
Statistical analysis
Statistical analysis was performed with SPSS 22 (IBM, Armonk, USA). Normal distribution of data was checked using the Shapiro-Wilk. Surface roughness (Ra), bacterial counts (logCFU) and buffering capacity (pH) were compared using t- or Mann-Whitney-U-test, or repeated measures analysis of variance (ANOVA). Generalized linear modeling (GLM) using the log-link was used to evaluate the association between loading, material and their interaction on mineral loss. The level of significance was set at p < 0.05.
2
Methodology
2.1
Study design
In the present study, miniature dentin-restoration specimens with simulated interfacial gaps were submitted to a cariogenic biofilm to induce secondary caries lesions. Concurrently, specimens were loaded occlusally, with different loads being applied. To test if the used restorative composite material moderated the association between loading and mineral loss, two different composites were employed: LEC- Low elastic modulus composite (Tetric Evo Flow, E-Modulus 5100 MPa, Ivoclar Vivadent, Schaan, Liechtenstein) and HEC- High elastic modulus composite (Tetric Evo Ceram, E-Modulus 10000 MPa, Ivoclar Vivadent, Schaan, Liechtenstein). While the elastic modulus of these composites was hypothesized to be the variable of interest for moderation of loading effects, we further characterized both materials for surface roughness on polished and non-polished surfaces, bacterial adhesion susceptibility, and buffering capacity (see Appendix A ). While both polished and non-polished surface roughness was lower for LEC than HEC, neither the bacterial adhesion susceptibility nor the buffering capacity was found to differ significantly between composites (see Appendix A ).
2.2
Specimens preparation
From 96 bovine incisors, 96 dentin slabs (2 × 3 × 6 mm) ( Fig. 1 a) were prepared (Band Saw 300cl, Exakt Apparatebau, Norderstedt, Germany) and polished (silicon carbide papers 1200, 2500, 4000 grit; Struers, Willich, Germany). Dentin slabs were embedded in methyl methacrylate resin (Technovit 4071, Heraeus Kulzer, Hanau, Germany) leaving the top and one side unprotected ( Fig. 1 b). Afterwards, specimens were randomly allocated to the two materials, LEC and HEC. On the top surface of the dentin specimens a self-etch adhesive (Scotchbond Universal, 3 M ESPE, St. Paul, USA) was applied leaving an unbonded U-shaped window (2 × 2 mm) ( Fig. 1 b). In this window, a 2 mm wide and 100 μm thick copper foil (Gemmel Metalle, Berlin, Germany) was placed, the first composite increment build up around the margin of the copper foil to fix it in place, and light-cured for 20 s (Valo, Ultradent, Salt Lake City, USA) at 1400 mW/cm 2 )( Fig. 1 c). A 2 mm thick composite layer was applied in bulk on the top surface of the dentin specimens and light-cured as described for 20 s ( Fig. 1 d). The copper foil was carefully removed, leaving a mean (SD) gap of 145 (25) μm between the composite and the dentin specimen ( Fig. 1 e). Gap size was not significantly different between any of the groups (p ≥ 0.05/t-test). The composite and dentin surfaces were finally polished with water-proof silicon carbide papers (4000 grit) and the patency of the gap was checked again using the copper foil.
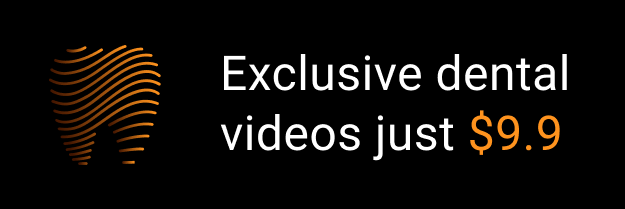