Highlights
- •
We develop new system for real-time measuring shrinkage stress and curing kinetics.
- •
The system shows unprecedented combination of sensitivity and resolution.
- •
Simultaneous measurement can be performed on composites with high filler content.
- •
Measurement of reaction exotherm during polymerization is justified.
- •
Mechanical constraint could affect polymerization kinetics.
Abstract
Objectives
Photopolymerized composites are used in a broad range of applications with their performance largely directed by reaction kinetics and contraction accompanying polymerization. The present study was to demonstrate an instrument capable of simultaneously collecting multiple kinetics parameters for a wide range of photopolymerizable systems: degree of conversion (DC), reaction exotherm, and polymerization stress (PS).
Methods
Our system consisted of a cantilever beam-based instrument (tensometer) that has been optimized to capture a large range of stress generated by lightly-filled to highly-filled composites. The sample configuration allows the tensometer to be coupled to a fast near infrared (NIR) spectrometer collecting spectra in transmission mode.
Results
Using our instrument design, simultaneous measurements of PS and DC are performed, for the first time, on a commercial composite with ≈80% (by mass) silica particle fillers. The in situ NIR spectrometer collects more than 10 spectra per second, allowing for thorough characterization of reaction kinetics. With increased instrument sensitivity coupled with the ability to collect real time reaction kinetics information, we show that the external constraint imposed by the cantilever beam during polymerization could affect the rate of cure and final degree of polymerization.
Significance
The present simultaneous measurement technique is expected to provide new insights into kinetics and property relationships for photopolymerized composites with high filler content such as dental restorative composites.
1
Introduction
Photopolymerized composites are widely used in applications ranging from electrical and optical materials, structural materials, coatings and adhesives, to various biomedical applications such as dental restoration. Photopolymerization exhibits several advantages over other polymerization techniques, including excellent spatial and temporal control, fast curing rates under ambient conditions, and solvent-free processing (non-toxic/green) . As monomers polymerize to form covalent bonds during the curing process, the volume of the resulting polymers can contract by significant amount, e.g. as high as 12% of the volume contraction for dental resins . Increasing the size or molecular mass of monomers, incorporating a larger amount of fillers, and tuning the reaction kinetics are some of the strategies to mitigate the adverse effects of polymerization shrinkage ; however, no approaches have been shown to fully eliminate polymerization shrinkage . Further, enormous polymerization stress (PS) can develop for materials that shrink by as little as 1% owing to the high modulus of these materials and can reduce the overall material performance. For example, in polymeric dental composites, considerable PS can develop at the tooth-composite interface that may lead to debonding, further tooth decay at the interface, and/or cracking of the tooth . In coatings and adhesives, stress generated may lead to surface cracking and debonding. One of the common features in photopolymerization processes is that gelation can take place very fast, e.g. less than a second under a high-intensity irradiation condition. Therefore, it is important to accurately follow the curing kinetics of such fast reactions for a better assessment of the performance of monomers, as well as understanding and controlling the curing process. The object of this study is to introduce new instrumentation that can continuously monitor the development of PS and curing kinetics in real-time for polymer composites with filler content as high as ≈80% by mass during photo-cure processing.
Polymerization shrinkage and stress are dictated by complex interplay among several factors, including curing kinetics (normally characterized by the degree of conversion, DC), development of resin shrinkage and modulus associated with DC, sample dimensions, and external constraint . Although there is generally a correlation between DC and PS, for practical purposes, DC is rarely used as a parameter to reduce PS since DC also correlates with other important material properties, e.g. mechanical properties , wear resistance , and the amount of leachable components . Several methods have been developed to characterize PS for photo-polymerized materials, including stress-strain-analyzers , universal testing machines (with an extensometer) with almost zero compliance , single cantilever devices with fixed compliance , and cantilever beam-based instruments (a tensometer) with adjustable compliance . To understand reaction kinetics and PS development, it is necessary to measure these properties simultaneously. It is known that slight changes in sample configuration, irradiation, or instrumental compliance can greatly affect the properties of complex photo-polymerizable systems. Therefore, there is a strong desire to combine these measurements for the same sample. To this end, Hsu et al. have demonstrated that Fourier Transform (FT) mid-infrared (mid-IR) spectroscopy can be coupled with a miniature cantilever system to effectively measure PS and DC simultaneously for photo-curing coatings . In their study, FT-IR was collected in reflection mode; therefore, only the immediate surfaces (top few microns) were measured . Later, near-IR (NIR) spectroscopy was shown to be capable of measuring double bond conversions in bulk samples in transmission mode. NIR exhibits lower molar absorptivity compared with the fundamental bands in the mid-IR region and shows two distinct peaks (6165 cm −1 and 4743 cm −1 ) for C C bond . Lu et al. showed that PS and DC for a limited range of composites could be determined simultaneously by combining NIR spectrometry with a tensometer. In that study, the dynamic range of the materials was limited by the instrument design (rigid beam), which reduced the sensitivity and accuracy of the instrumentation . This design also required a sample with diameter of ≈6 mm, which was too large for accurate collection of transmission NIR at high filler contents due to the absorption and attenuation of the IR signal intensity. Using this instrument design, Lu et al. were able to measure composites with up to 30% by mass filler content . Many practical applications use a significantly higher amount of fillers (as high as 80% by mass ) to improve the mechanical, tribological, and thermal properties of the materials .
In this study, a tensometer constructed according to our design criteria was coupled to a high-speed data acquisition NIR spectrometer to enable real-time simultaneous measurement of PS and DC for highly filled polymer composites. Since temperature rise during photo-polymerization is usually a critical parameter in determining the applicability of the material, and reaction exotherm is also associated with the curing kinetics, the evolution of exothermic temperature is measured in parallel. Due to the existence of a vast number of monomer systems, studies related to methacrylate-based composites were chosen here. A capacitive displacement sensor and data acquisition devices/programs were incorporated in our tensometer system to improve the resolution and acquisition speed of the curing kinetics. Our results indicate that the simultaneous measurement can be performed on a model highly-filled composite (≈80% by mass) at a high acquisition rate in excess of 10 data points per second, which gives sufficient data to accurately evaluate curing kinetics from the inception of polymerization. This higher resolution instrument also provides additional insight of curing kinetics under different degrees of constraint (achieved by adjusting sample position along the cantilever-beam of the tensometer). The null hypothesis tested was that changing the degree of constraint would have no effect on the curing kinetics, reaction exotherm, and final degree of polymerization.
2
Materials and experiments 1
1 Certain commercial materials and equipment are identified in this manuscript in order to specify adequately the experimental and analysis procedures. In no case does such identification imply recommendation or endorsement by the National Institute of Standards and Technology (NIST) nor does it imply that they are necessarily the best available for the purpose.
In this investigation, a commercial dental composite (TPH Spectra™, Dentsply-Caulk, Milford, DE) was used as the test material to demonstrate the capabilities of the newly developed system for evaluating the development of PS and DC. TPH is a blue-light activated, urethane modified Bis-GMA/TEGDMA (50:50 mass ratio) based composite, filled primarily with barium boron aluminum silicate glass particles at 78% by mass (≈57% by volume).
A cantilever beam-based instrument (tensometer) presented in our previous study has been further improved (described below) and a dispersive NIR spectrometer has been coupled to the instrument as shown in Fig. 1 a. Using this device, an uncured composite (or resin) specimen was bonded with two quartz rods, the lower rod was fixed and the upper rod attached to a cantilever beam. Upon photo-polymerization, the composite shrinkage stress induced a deflection in the calibrated cantilever beam, which was recorded by a displacement sensor at the free end of the beam. The PS was then calculated through the beam formula :
where σ is the PS and F is the force exerted by the sample shrinkage (see schematic in Fig. 1 c); A and r are the cross-sectional area and the radius of the sample, respectively; δ is the beam deflection at the free end; E and I (= wh 3 /12) are the Young’s modulus and the moment of inertia of the beam, respectively; h and w are the height and width of the beam cross-section, respectively; l and a are the length of the beam and the distance between the sample position and the clamped edge of the beam, respectively.
Instead of using a linear variable differential transformer (LVDT) as previous studies , a capacitive displacement sensor with 20-nm resolution (Driver Model: CPL190, Probe Model: C18-13, Lion Precision, St. Paul, MN) was used to provide more sensitivity for detecting the onset of shrinkage development (pre-gel stage). The analog data detected by the sensor were collected using the LabView software program (National Instruments, Austin, TX) via a 24-bit DAQ device (USB-2404-10, Measurement Computing, Norton, MA). Additionally, two micro stages with center aperture (GO ® , Edmund Optics, Barrington, NJ) were assembled onto the base stand in X-Y configuration with the bottom quartz rod passing through their apertures, such that the alignment of the top and bottom rods can be easily achieved.
The remote NIR data acquisition was realized by configuring two optical-fiber cables (1 mm diameter, Multimode Fiber Optics, Inc., Hackettstown, NJ) onto the sides of the sample, with one cable connected to the IR light source (LS-1, Ocean Optics, Inc., Dunedin, FL) and the other to the NIR spectrometer (NIRQuest512-2.2, Ocean Optics, Inc., Dunedin, FL; Fig. 1 b for a close view of the sample mounting region). The NIRQuest is a dispersive infrared spectrometer with a built-in diffractive grating-based optical bench, a 512-element InGaAs linear-array detector, and a 16-bit USB A/D converter. Although the dispersive spectrometer possesses relatively lower resolution, lower signal-to-noise ratio, and narrower spectral range than the bench top FT-IR instruments used in previous studies , it can provide much higher data acquisition rate and is more portable than FT-IR. The latter involves the movement of the interferometer optics and also the overhead associated with the computer processing of the Fourier transform of the raw data. The scan rate of the system used here is up to 1000 Hz (integration time from 1 ms to 120 s) over the spectral range of 900 nm to 2200 nm (4500 cm −1 to 11,100 cm −1 ).
Simultaneous measurement of PS and DC were carried out using the tensometer and the NIR spectrometer as described above. The diameter and height of the composite sample were 2.5 mm and 2 mm, respectively. A non-tacky polytetrafluoroethylene (PTFE) sleeve (inner diameter: 2.5 mm, wall thickness: 0.4 mm) with an injection hole and a smaller air-venting hole was used to encase the rods and composite sample. The curing lamp (QHL75™ curing light, Dentsply-Caulk, Milford, DE) was attached to the lower rod with an opaque flexible light guide (10 mm diameter). The intensity of the curing light, measured by a Cure Rite radiometer (model #8000, EFOS Inc., Williamsville, NY), was (500 ± 10) mW/cm 2 at the upper end of the bottom rod where the sample was bonded and took ≈0.4 s to reach the maximum after switch on. The irradiation start and end times were controlled by the LabView program through a controllable power outlet, such that the data collection and the photo-activation could be synchronized. For our measurements, the curing lamp was switched on at 20 s after the start of data collection and off at 60 s ( i.e. , 40 s irradiation). According to the material manufacturer, this amount of irradiation was more than enough to cure a 2 mm thick sample that adopted in this study. The PS was recorded at a rate of 10 data points per second. The average of the data collected during the first 20 s (prior to irradiation) is used as the zero baseline. The NIR spectra were collected continuously at an integration time of 85 ms, which was also synchronized with the PS collection process. This optimal integration time was determined based on a series of test runs, which showed spectra of sufficient resolution and minimized noise. For the calculation of DC, the absorption peak of the methacrylate functional group centered at 6165 cm −1 (C C H stretching, first overtone) was used. The area under this peak was determined by subtracting a linear baseline across the edges of the peak for each spectrum obtained. Small variations in the intensity of the baseline were compensated for since the peak area was always calculated relative to the baseline of each individual spectrum. The DC was calculated by taking the peak area of the sample prior to the start of irradiation (Area monomer ) and at each point during the polymerization process (Area polymer ) based on the following formula :
To compare the spectra obtained by the dispersive NIR (D-NIR) and those by FT-IR in terms of resolution and acquisition rate, the uncured resin disks were measured using both instruments (FT-IR used: Nexus 670, XT-KBr beam splitter and MCT/A detector, Nicolet Instrument, Madison, WI), respectively. The FT-IR spectra (4500–6500 cm −1 ) were collected through a NIR fiber port (Smart Fiberport, Nicolet Instrument, Madison, WI) with a 2 scans per spectrum and 8-wavenumber resolution (the typical parameters for real-time monitoring used in the literature), and also a 64 scans and 8 wavenumber resolution. To explore the effect of external constraint on the curing process, simultaneous measurements were performed when the upper rod was fixed at two different cantilever beam positions representing the higher and lower end of beam length ( i.e. 6 cm and 20 cm from the fixed end of the cantilever-beam), such that the rigidity of the effective beam experienced a near 40-fold change.
Experiments were conducted under a yellow light environment to minimize premature photopolymerization and at room temperature. The temperature increase due to the exothermic polymerization was also monitored at 10 Hz with a K-type miniature thermocouple (0.5 mm diameter, Omega Engineering Inc., Stamford, CT) embedded into the very edge (to avoid affecting the sample integrity) of the sample through the injection hole of the PTFE sleeve. In doing so, only temperature of the sample periphery was measured. For each set of experiments, three replicates were conducted.
2
Materials and experiments 1
1 Certain commercial materials and equipment are identified in this manuscript in order to specify adequately the experimental and analysis procedures. In no case does such identification imply recommendation or endorsement by the National Institute of Standards and Technology (NIST) nor does it imply that they are necessarily the best available for the purpose.
In this investigation, a commercial dental composite (TPH Spectra™, Dentsply-Caulk, Milford, DE) was used as the test material to demonstrate the capabilities of the newly developed system for evaluating the development of PS and DC. TPH is a blue-light activated, urethane modified Bis-GMA/TEGDMA (50:50 mass ratio) based composite, filled primarily with barium boron aluminum silicate glass particles at 78% by mass (≈57% by volume).
A cantilever beam-based instrument (tensometer) presented in our previous study has been further improved (described below) and a dispersive NIR spectrometer has been coupled to the instrument as shown in Fig. 1 a. Using this device, an uncured composite (or resin) specimen was bonded with two quartz rods, the lower rod was fixed and the upper rod attached to a cantilever beam. Upon photo-polymerization, the composite shrinkage stress induced a deflection in the calibrated cantilever beam, which was recorded by a displacement sensor at the free end of the beam. The PS was then calculated through the beam formula :
where σ is the PS and F is the force exerted by the sample shrinkage (see schematic in Fig. 1 c); A and r are the cross-sectional area and the radius of the sample, respectively; δ is the beam deflection at the free end; E and I (= wh 3 /12) are the Young’s modulus and the moment of inertia of the beam, respectively; h and w are the height and width of the beam cross-section, respectively; l and a are the length of the beam and the distance between the sample position and the clamped edge of the beam, respectively.

VIDEdental - Online dental courses
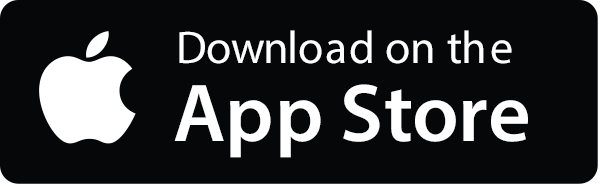
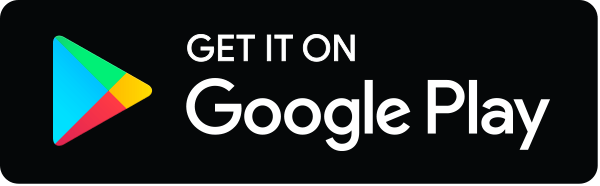