Introduction
Orthodontic tooth movement (OTM) relies on efficient remodeling of alveolar bone. While a well-controlled inflammatory response is essential during OTM, the mechanism regulating inflammation is unknown. Autophagy, a conserved catabolic pathway, has been shown to protect cells from excess inflammation in disease states. We hypothesize that autophagy plays a role in regulating inflammation during OTM.
Methods
A split-mouth design was used to force load molars in adult male mice, carrying a GFP-LC3 transgene for in vivo detection of autophagy. Confocal microscopy, Western blot, and quantitative polymerase chain reaction analyses were used to evaluate autophagy activation in tissues of loaded and control molars at time points after force application. Rapamycin, a Food and Drug Administration–approved immunosuppressant, was injected to evaluate induction of autophagy.
Results
Autophagy activity increases shortly after loading, primarily on the compression side of the tooth, and is closely associated with inflammatory cytokine expression and osteoclast recruitment. Daily administration of rapamycin, an autophagy activator, led to reduced tooth movement and osteoclast recruitment, suggesting that autophagy downregulates the inflammatory response and bone turnover during OTM.
Conclusions
This is the first demonstration that shows that autophagy is induced by orthodontic loading and plays a role during OTM, likely via negative regulation of inflammatory response and bone turnover. Exploring roles of autophagy in OTM holds great promise, as aberrant autophagy is associated with periodontal disease and its related systemic inflammatory disorders.
Highlights
- •
Autophagy is activated in peri-dental tissues by orthodontic loading.
- •
Autophagic activity correlates with inflammatory markers after force application.
- •
Rapamycin, an autophagy inducer, reduces tooth movement and osteoclast recruitment.
- •
Data suggest autophagy decreases inflammation and bone turnover after tooth loading.
Orthodontic tooth movement (OTM) combines bone remodeling with reversible periodontal injury due to mechanical strain. Under healthy conditions, movement is achieved by coordinated bone adaptation, involving osteoclast resorption of compressed bone and osteoblast bone formation under tension. This process is regulated by an aseptic inflammatory response characterized by release of inflammatory mediators, such as prostaglandins and cytokines. Inflammation leads to vasodilation and permeability, prompting leukocyte migration into paradental extracellular matrix. Migratory leukocytes, fibroblasts, and osteoblasts, activate an inflammatory cascade through chemokines and cytokines. Cytokines act on periodontal ligament (PDL) cells, promoting osteoclastogenesis through upregulation of Receptor Activator of Nuclear Factor Kappa-B Ligand (RANKL). Increased RANKL combined with decreased osteoprotegerin (OPG) release by osteoblasts favors osteoclast differentiation and bone resorption. Release of cytokines Interleukin-1β (IL-1β) and Tissue Necrosis Factor-α (TNFα) induce osteoclast differentiation, function, and survival. At the tension side, Interleukin-10 (IL-10) level is increased, boosting OPG and reducing RANKL production by osteoblasts, which favors bone deposition through inhibition of osteoclast formation. Transforming growth factor-β level is also elevated under tension, which recruits PDL cells and differentiating osteoblast precursors. ,
Extensive studies have dissected OTM signaling cascades, but little is known about how inflammation is regulated during OTM. In healthy patients, load-induced inflammation is well-controlled, with PDL space remaining fairly constant. Inflammation resolves and tissues return to homeostasis, but it is unknown how inflammation is downregulated after OTM. To identify potential regulatory mechanisms, we are investigating the role of autophagy, an evolutionarily conserved self-protective modulator of inflammation. , Defective autophagy contributes to diseases with chronic or dysregulated inflammation such as periodontitis, systemic lupus erythematosis, and inflammatory bowel disease. Functional autophagy modulates inflammation-inducing processes for proper resolution of systemic disease ; we aim to explore if autophagy regulates OTM through a similar mechanism.
The most studied form of autophagy is macroautophagy, henceforth referred to as autophagy. Autophagy is an intracellular catabolic pathway induced by stressful conditions including starvation, hypoxia, toxin accumulation, or damaged organelles. During normal conditions, UNC-51-like kinase 1 (ULK1) is negatively regulated by mammalian and mechanistic target of rapamycin (mTOR), but under stress, AMP-activated protein kinase inhibits mTOR and phosphorylates ULK1, initiating autophagy ( Fig 1 ). , Activation results in autophagosome formation, a double-membraned organelle that sequesters damaged cytosolic components. Phagophore formation begins with the Beclin-1/VPS34 class III PI3K complex, followed by ATG protein conjugation, LC3 membrane insertion, and capture of targets for degradation ( Fig 1 ). The mature autophagosome then fuses with the lysosome; sequestered contents with cargo receptor p62 are broken down and released back to the cytosol for reuse. ,

In addition to canonical roles in homeostasis and cell survival, autophagy is important in host defense through crosstalk with inflammation. Autophagy can modulate inflammation through regulation of proinflammatory cytokines and inhibition of inflammasome activation and production of IL-1β and IL-18. , , Th1 type/proinflammatory cytokines IL-1β and TNFα induce autophagy, whereas Th2-type cytokines IL-4, IL-10, and IL-13 suppress autophagy induction by activating mTOR. ,
Furthermore, a growing literature ties autophagy to bone turnover. Microgravity induces autophagic activity in pre-osteoclasts prompting differentiation.
Murine studies in rheumatoid arthritis show TNFα induces autophagy in osteoclasts, whereas activation of autophagy through Beclin-1 overexpression promotes osteoclastogenesis and resorption. Nollet et al found autophagy induced in osteoblasts during mineralization under oxidative stress. Osteoblasts with defective autophagy show increased oxidative stress and secretion of RANKL, favoring generation of osteoclasts and bone resorption; osteoblast-specific autophagy-deficient mice lost half their trabecular bone mass.
With mounting evidence of crosstalk between autophagy, inflammation, and bone metabolism, we aimed to investigate roles of autophagy in regulating the orthodontically induced inflammatory response and bone turnover using an established mouse model of OTM. Basal levels of autophagy are observed in dental pulp and odontoblasts during homeostasis and autophagy markers, LC3 and Beclin-1, are expressed throughout odontogenesis. Autophagy is active in the developing and adult dentition, yet our knowledge of its roles is highly limited. Using a transgenic mouse model with a fluorescently tagged autophagy protein LC3, we are the first group to demonstrate autophagy activation in response to mechanical loading in vivo. This is a critical first step to exploring roles of autophagy in OTM, which holds great promise, as aberrant autophagy is associated with periodontal disease and its related systemic inflammatory disorders, diabetes, and cardiovascular diseases. , We hypothesize that autophagy is induced in peri-dental tissues in response to orthodontic loading of teeth and regulates load-induced inflammation and bone turnover.
Material and methods
Orthodontic force application is an established murine model in OTM studies. Optimal conditions have been characterized. Mice were anesthetized by intraperitoneal injection of xylazine (10 mg/kg) and ketamine (100 mg/kg) solution. We employed a split-mouth design, where 30 g of force (= 0.3 N) was delivered to the maxillary right first molar in the mesial direction, by bonding a nickel-titanium (NiTi) closed coil spring (American Orthodontics, Cat# 855-181, length adapted to each mouse’s mouth) between the maxillary right first molar and incisors with light-cured resin (Transbond Supreme LV, 3M Unitek, Morovia, Calif) ( Fig 2 , A – C ). No spring reactivation was performed after bonding. All animal care procedures followed the ethical regulations for animal experiments, defined by Institutional Animal Care and Use Committee of the University of North Carolina at Chapel Hill. All mice were monitored daily and given softened food after spring loading; no significant weight loss occurred during the experiment period.

For histomorphometric analyses, that is OTM distance measurement, detection of autophagy activity, and tartrate-resistant acid phosphatase (TRAP) staining, we used a GFP-LC3 reporter mouse line. , GFP-tagged LC3 is expressed under the LC3 promoter and inserted into the autophagosome membrane with autophagy induction ( Fig 1 ) , ; this yields green fluorescent puncta throughout the cytoplasm, visible with confocal microscopy ( Fig 2 ). An increase of intracellular LC3-autophagosome puncta indicates autophagy induction or downstream suppression, which was imaged and quantified to measure autophagy activity in vivo.
Orthodontic force application in mice using a split-mouth design is a well-accepted model ( Fig 2 , A – C ). Thirty GFP-LC3 adult male mice (8-9 weeks old, in C57BL/6 background) were subdivided into 6 groups ( n = 5 for each group) for sacrifice at 6 different time points (days 0, 1, 3, 5, 7, and 10) after spring loading.
For molecular analyses, that is mRNA/quantitative real-time polymerase chain reaction (qRT-PCR) and Western Blot, 60 wild-type adult male mice (C57BL/6, 8-9 weeks old, obtained from Jackson Laboratory, Bar Harbor, Mai) were subdivided into 6 groups, to be killed at 6 different time points (days 0, 1, 3, 5, 7, and 10). Half of each group ( n = 5) was used for mRNA/qRT-PCR and half ( n = 5) for Western Blot experiments.
For rapamycin injection experiments, intraperitoneal injection of rapamycin (6 mg/Kg/d) or rapamycin vehicle (control) solution were given to mice daily beginning on the day of spring placement and until the mice were killed. The half-life of rapamycin in mouse blood was found to be 15 hours. Rapamycin was initially injected at a dosage of 8 mg/kg/d as previously described ; however, at this dose, our experimental mice experienced toxicity demonstrated by lethargy and weight loss, compared with the control vehicle group. As a result, we titrated lower rapamycin dosages and found 6 mg/kg/d was well-tolerated by mice. Rapamycin solution was made as previously described. Briefly, a 20 mg/ml stock solution of rapamycin (LC Laboratories, Woburn, Mass) was prepared in ethanol. The stock solution was diluted to 1.2 mg/ml in vehicle solution (saline containing 5% polyethylene glycol 400 and 5% Tween-80). The vehicle solution was prepared under the same conditions without inclusion of rapamycin. Sixty GFP-LC3 adult male mice (8-9 weeks old, in C57BL/6 background) were subdivided into 6 groups ( n = 10 for each group, half injected with rapamycin and half injected with rapamycin vehicle solution), and were killed at different time points (days 1, 3, 5, 7, and 10) after spring loading. For molecular analysis, 10 wild-type adult male mice (C57BL/6, 8-9 weeks old, from Jackson Laboratory), half injected with rapamycin and half injected with rapamycin vehicle solution, were killed 1 day postspring loading for mRNA/RT-PCR experiment.
The occlusal view of the maxilla was imaged using a stereomicroscope (SMZ18, Nikon Instruments, Melville, NY) with an adapted digital camera (Nikon Instruments). NIS-Elements Basic Research imaging software was used for distance measurements, by measuring the distance between 2 parallel lines tangent to the convex regions distal to the first molar and mesial to the second molars ( n = 5 for each time point). Both the experiment (E) and control (C) sides were measured. The OTM distance measurement of the maxillary right first molar was determined by subtracting the measurement of the C unloaded side measurement from the E loaded side (OTM distance = E side value − C side value).
To confirm measurements taken on the stereomicroscope, microcomputed tomography (microCT) radiographs were taken at days 1, 5, and 10 postloading. Mice maxillae were harvested at each time point (days 1, 5, and 10) and immediately fixed in 10% neutral formalin solution for 3 days. MicroCT radiographs of the maxillae were acquired using Skyscan 1275 (Skyscan, Aartselaar, Belgium) with the X-ray source set at 50 kV and 200 μA. Each sample was rotated 360 ° and imaged every 0.1 ° at 8 μm image pixel size, with an exposure time of 35 ms and averaging of 3 frames per view. Two-dimensional images from the bucco-lingual midpoint of the first and second molar were extracted from the 3-dimensional volumetric file. MicroCT 2-dimensional images and measurements were taken at the height of contour between the first and second molars.
Adult male mice were killed with CO 2 asphyxiation. Tissue preparation procedures were conducted as previously described with modification. Maxillae including part of the scalp were dissected free of adherent tissue and placed in a processing/embedding cassette (Fisher Scientific, Cat #15-182-706). Samples were fixed in fresh 4% paraformaldehyde (4 ° C, 3 days), followed by decalcification in 14% EDTA solution (4 ° C, 4 days). After ×1 phosphate buffered saline (PBS) washes (1-2 hours, room temperature), samples were equilibrated in 30% sucrose ×1 PBS solution overnight at 4 ° C. Each maxilla was bisected and embedded in OCT compound (Fisher Healthcare, Cat # 23-730-571) containing base mold (Fisherbrand, Cat # 22-363-553). Embedding media was flash frozen on a metal platform prechilled in a dry ice-ethanol bath. Molds were wrapped in aluminum foil and stored at –80 ° C. Cryosectioning (6 μm thickness) was performed on a Leica CM 1520 (Leica Biosystems, Wetzlar, Germany), and sections were collected consecutively on Superfrost Plus slides (Fisherbrand, Cat # 22-037-246). Slides were stored at –20 ° C.
For GFP imaging, frozen sections were washed with ×1 PBS and nuclei were counterstained with DAPI (Sigma-Aldrich, Cat # D9542-10MG). Slides were mounted using VectaShield Mounting Medium (Vector Laboratories, Cat # H-1000) with coverslips. Sections were imaged on Zeiss LSM 710 Laser Scanning Confocal microscopes. Images were analyzed using Imaris (Bitplane) and Photoshop (Adobe). A trained technician reviewed each image at a uniform magnification and counted intracellular puncta and nuclei in a fixed rectangular area overlaid on the image. Puncta were defined as small, discrete points of green fluorescence visible within the cytoplasm, distinct from other intracellular structures.
TRAP staining was performed as described. Sections were counterstained with Mayers/Harris Hematoxylin for 1-2 minutes, dehydrated, and mounted with coverslip using VectaShield Hard Set mounting medium. Images were obtained with a Nikon Eclipse Ti-U inverted microscope, using NIS-Elements Basic Research imaging software (Nikon Instruments Inc). Brightfield was used for cellular visualization at ×20 magnification with an average exposure time of 100 ms.
RNA extraction and qRT-PCR were performed for each animal individually ( n = 5 at all time points). After sacrifice, maxillary first molars and their peri-dental tissues (PDL and alveolar bone) were extracted; because of the miniscule size of mouse molars and the resulting inability to separate peri-dental tissues from the root and from each other, tissue was processed in total. Tissue total RNA was isolated with TRIzol (Invitrogen) according to manufacturer’s instructions. The resultant DNA-free RNA was diluted in RNase-free water and quantified by the Nanodrop (Thermo) at 260 nm. Total RNA was reverse transcribed using iScript cDNA Synthesis kit (Bio-Rad, Cat # 170-8891). The iTaq Universal SYBR Green Supermix Kit (Bio-Rad, Cat # 172-5120) was used for quantitative real-time RT-PCR analysis. Primers were designed using Primer Express (Applied Biosystems) and synthesized by Invitrogen ( Table I ). Genes assayed included inflammatory cytokines (TNFα, Il-6, Il-1β, and NFATC1), bone turnover markers (RANKL, OPG, and MMP9) and autophagy pathway genes ( Becn1 , LC3 , and Atg5 ). Relative differences in gene expression between groups were determined from cycle time (Ct) values. The values were normalized to beta-2-microglobulin (B2M, an internal positive control) in the same sample (ΔCt) and expressed as fold-change over day 0 control (2 −ΔΔCt ). Real-time fluorescence detection was carried out using an ABI StepOnePlus Real-time PCR system (Applied Biosystems).
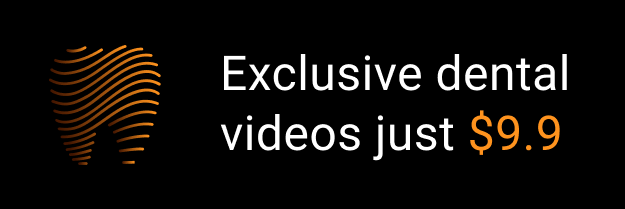