Highlights
- •
Real time imaging of the two dimensional (2D) axial shrinkage field in the bonded disk geometry is presented for two resin-based composites (RBCs).
- •
The time-dependent 2D axial shrinkage field depends strongly upon the light curing unit beam profile, sample geometry, and RBC visco-elastic properties.
- •
For a uniform beam profile and conventional sample geometry, the axial 2D shrinkage strain is uniform to within 4% over the central part of the sample.
Abstract
Objective
To investigate the time evolution of the two dimensional axial shrinkage field for two dental resins in the bonded disk geometry and further test the bonded-disk method.
Methods
An interferometric technique employing a camera was used to image the 2D axial shrinkage field when polymerizing dental resins in real time both during and after light exposure. Four different beam profiles from two light curing units and three sample geometries were utilized to investigate their roles on the 2D axial shrinkage field.
Results
The 2D axial shrinkage field correlates qualitatively with the beam profile shortly after the start of light exposure but takes on distinct shapes caused by the rigidity of the coverslip, beam profile, and the resin viscoelasticity.
Significance
Using the conventional bonded disk geometry and uniform beam profile from a light curing unit, the axial 2D shrinkage field was uniform to within 4% in the central part of bonded disk samples.
1
Introduction
Along with the Young’s modulus of elasticity and hardness, the shrinkage strain induced in photocured resin-based composites (RBCs) is one of their fundamental properties . Upon shrinkage, the RBC material placed in the tooth cavity pulls on the semi rigid tooth walls resulting in large built in stress within the tooth. With time, microcracking and marginal gaps may develop along the interface between the RBC and tooth walls resulting in microleakage, secondary caries, and clinical failure of the restoration .
Numerous techniques have been developed and applied to quantify the shrinkage strain in photo-polymerized RBCs. The volumetric shrinkage was evaluated using dilatometry where the minute decrease in volume due to curing of a RBC sample in a liquid was measured . A second technique employing Archemide’s principle was applied where the change in weight between the uncured and cured RBC sample in air and submerged in a liquid is used to estimate the volumetric shrinkage .
Other techniques focussed on measuring the axial shrinkage strain in RBCs. Wilson introduced a deflecting blade technique involving a flexible steel blade overlying a specimen disk. This was used to detect the onset of the rigid contraction stage of polymerization after the ‘post-gel’ time point, rather than the total contraction, especially for self-curing composites. Watts et al. and others further developed this approach now known as the “Bonded Disk method”, for measurement of total shrinkage from time zero, with supplementary analysis by Lee et al. . This is now readily employed worldwide due to its ease of use .
The technique consists of measuring the axial shrinkage of a thin disk shaped RBC sample adhered between a rigid glass substrate and flexible glass coverslip supported on its edge by a metal ring. Due to the strong bonding between the RBC and glass and large sample diameter to thickness ratio (>5) used, the in plane shrinkage is greatly suppressed and the volumetric shrinkage becomes approximately equal to the axial shrinkage. The axial shrinkage is measured by monitoring the coverslip deflection at the center of the sample using a mechanical position sensor that is in direct contact with the coverslip.
Conventional optical, interferometric, and imaging techniques have also been applied to determine the shrinkage at a point on the surface or across the surface of RBC samples . In a recent study , a camera was used to image the positions of fluorescent tracer particles on the surface of a RBC sample before, during, and after light exposure from a light curing unit (LCU). As the RBC sample polymerized with time, the particles attached to the surface followed the sample surface shrinkage. The time dependent shrinkage field was reconstructed from the position of the tracer particles recorded at each camera frame. To obtain a sufficiently high spatial resolution on the sample (<4.54 μm/pixel), a camera with a high 6.0 Megapixels density was required. However, the high pixel density of the sensor limited the data collection to only two frames per second during RBC polymerization.
Recently, Kostylev et al. combined the sample geometry of the bonded-disk method with the high axial resolution obtained using an interferometer with a HeNe laser source and high spatial resolution of an imaging camera system to measure, without contacting the sample, the axial shrinkage across the entire face of a disk shaped RBC sample in real time before, during, and after light exposure with a temporal and spatial resolution of 8 ms and 21 μm, respectively. They showed that for a dental resin exposed to light from a LCU with a ‘turbo’ light guide, the shrinkage map evolved from a flat surface to a conical shape surface to a final “M”–like shape near the center of the sample. These results were unexpected as it was previously shown that a RBC sample surface after curing was measured to be flat using stylus-based profilometry and, consequently, all determinations of the volumetric shrinkage strain in RBCs using this method are derived from measurements of the shrinkage at a single point at the center of the samples.
In this study, this technique is applied to investigate the 2 dimensional (2D) axial shrinkage field in real time as a function of the LCU beam profile and sample geometry. The null hypotheses were: (1) there is no correlation between the beam profile and 2D shrinkage field, and (2) there is no correlation between the sample geometry and 2D shrinkage field.
2
Materials and methods
2.1
Method for measuring the 2D axial shrinkage field
The bonded-disk method was used to determine the axial shrinkage of a dental resin. However, instead of utilizing a position sensor to measure the deflection at the center of the sample, a 10 mm diameter HeNe laser beam was employed to monitor the sample deflection across its whole surface. In brief, the sample was used as the moving mirror of a Michelson interferometer. The modulated laser beam exiting the interferometer was focused onto the CCD sensor of a camera. A series of interference fringes formed on the camera sensor were recorded at 122 frames per second before, during and after light exposure from the LCU. A custom program analyzed the interference pattern data over the central 9 mm diameter region of the sample to reconstruct the shrinkage topography with time. A detailed system description, calibration, and typical data are reported elsewhere .
2.2
Sample preparation and geometries
Two RBCs were used in this study; Filtek™ Supreme Ultra CT (CT) produced by 3 M ESPE and Tetric™ EvoCeram A3 (EC) made by Ivoclar Vivadent Inc. The CT product contains only camphorquinone (CQ) while the dental resin EC contains both CQ and monoacylphosphine oxide (Lucirin® TPO) whose peak sensitivities in their absorption spectra are at 470 nm and 381 nm, respectively .
The bonded disk sample configuration was used to measure the surface topography of a RBC. A disk shaped RBC sample of 1.22 mm thickness and 9 mm diameter was placed on a 3 mm thick quartz plate of 25.4 mm diameter. The quartz substrate was sanded using 1200 grit SiC sand paper and then silanized to increase its bonding with the RBC sample. A brass ring of 20 mm inner and 24 mm outer diameter was used to define the sample thickness. The brass ring’s thickness was 1.22 mm thick. A coverslip was placed on top of the RBC sample to make it flat and acted as a reflecting surface for the laser beam. Three sample geometries were utilized to investigate the role of the coverslip in the 2D shrinkage field. Geometry No. 1 consisted of the standard sample configuration used elsewhere described above and with a 100 μm thick Borosilicate glass coverslip of 24 mm diameter and a brass ring (G + R). Geometry No. 2 was the same as No. 1 except that the brass ring was removed (G) before light exposure. Geometry No. 3 was the same as No. 2 except that the glass coverslip was replaced by a 25 μm thick circular Polyethylene Terephthalate (Mylar™) disk (M) of 24 mm diameter. Note that the glass coverslip was not silanized.
During the course of the experiments, the sample temperature varied from 20.7 °C to 23.9 °C with a corresponding mean temperature of 22.3 °C. The latter temperature will be quoted for all the results.
2.3
LCU irradiance beam profiles and power measurements
Four different irradiance beam profiles ranging from highly uniform to highly non-uniform profiles were used in this study. A Plasma Arc (PAC) unit (Sapphire, Den-Mat Holdings, Santa Maria, CA) and a LED-based two emission wavelengths (polywave®) unit with its original light guide (Bluephase Style LCU, Ivoclar Vivadent Inc., Amherst, NY, USA) were used for this study. The PAC unit was fitted with either a reverse turbo 6.7/11.6 mm light guide, referred below as a standard light guide, or turbo 6.1/2.5 mm light guide. A third light guide configuration was obtained by using the standard light guide fitted with a 250 μm diameter aperture attachment at the tip end of the light guide. The irradiance beam profiles were measured through a 3 mm thick quartz plate using a commercial beam profiler (LBA-USB-L070 Beam Profiler, Ophir-Spiricon, Logan, UT, USA) and is described elsewhere . The total power output from the PAC unit with each of the three light guide configurations and LED-based polywave® unit were measured through a 3 mm thick quartz plate using a calibrated thermopile and meter (PM-10 detector and FieldMax meter, Coherent Inc., Santa Clara, CA, USA) .
2.4
Statistical methods
Three samples were photo-cured and analyzed for each sample geometry and beam profile except for sample geometry No. 1 and turbo light guide configuration where two samples were studied. Two-way ANOVA and Protected Least Significant Difference (PLSD) Fisher’s tests were applied to evaluate statistical differences in the data collected under different experimental conditions.
2
Materials and methods
2.1
Method for measuring the 2D axial shrinkage field
The bonded-disk method was used to determine the axial shrinkage of a dental resin. However, instead of utilizing a position sensor to measure the deflection at the center of the sample, a 10 mm diameter HeNe laser beam was employed to monitor the sample deflection across its whole surface. In brief, the sample was used as the moving mirror of a Michelson interferometer. The modulated laser beam exiting the interferometer was focused onto the CCD sensor of a camera. A series of interference fringes formed on the camera sensor were recorded at 122 frames per second before, during and after light exposure from the LCU. A custom program analyzed the interference pattern data over the central 9 mm diameter region of the sample to reconstruct the shrinkage topography with time. A detailed system description, calibration, and typical data are reported elsewhere .
2.2
Sample preparation and geometries
Two RBCs were used in this study; Filtek™ Supreme Ultra CT (CT) produced by 3 M ESPE and Tetric™ EvoCeram A3 (EC) made by Ivoclar Vivadent Inc. The CT product contains only camphorquinone (CQ) while the dental resin EC contains both CQ and monoacylphosphine oxide (Lucirin® TPO) whose peak sensitivities in their absorption spectra are at 470 nm and 381 nm, respectively .
The bonded disk sample configuration was used to measure the surface topography of a RBC. A disk shaped RBC sample of 1.22 mm thickness and 9 mm diameter was placed on a 3 mm thick quartz plate of 25.4 mm diameter. The quartz substrate was sanded using 1200 grit SiC sand paper and then silanized to increase its bonding with the RBC sample. A brass ring of 20 mm inner and 24 mm outer diameter was used to define the sample thickness. The brass ring’s thickness was 1.22 mm thick. A coverslip was placed on top of the RBC sample to make it flat and acted as a reflecting surface for the laser beam. Three sample geometries were utilized to investigate the role of the coverslip in the 2D shrinkage field. Geometry No. 1 consisted of the standard sample configuration used elsewhere described above and with a 100 μm thick Borosilicate glass coverslip of 24 mm diameter and a brass ring (G + R). Geometry No. 2 was the same as No. 1 except that the brass ring was removed (G) before light exposure. Geometry No. 3 was the same as No. 2 except that the glass coverslip was replaced by a 25 μm thick circular Polyethylene Terephthalate (Mylar™) disk (M) of 24 mm diameter. Note that the glass coverslip was not silanized.
During the course of the experiments, the sample temperature varied from 20.7 °C to 23.9 °C with a corresponding mean temperature of 22.3 °C. The latter temperature will be quoted for all the results.
2.3
LCU irradiance beam profiles and power measurements
Four different irradiance beam profiles ranging from highly uniform to highly non-uniform profiles were used in this study. A Plasma Arc (PAC) unit (Sapphire, Den-Mat Holdings, Santa Maria, CA) and a LED-based two emission wavelengths (polywave®) unit with its original light guide (Bluephase Style LCU, Ivoclar Vivadent Inc., Amherst, NY, USA) were used for this study. The PAC unit was fitted with either a reverse turbo 6.7/11.6 mm light guide, referred below as a standard light guide, or turbo 6.1/2.5 mm light guide. A third light guide configuration was obtained by using the standard light guide fitted with a 250 μm diameter aperture attachment at the tip end of the light guide. The irradiance beam profiles were measured through a 3 mm thick quartz plate using a commercial beam profiler (LBA-USB-L070 Beam Profiler, Ophir-Spiricon, Logan, UT, USA) and is described elsewhere . The total power output from the PAC unit with each of the three light guide configurations and LED-based polywave® unit were measured through a 3 mm thick quartz plate using a calibrated thermopile and meter (PM-10 detector and FieldMax meter, Coherent Inc., Santa Clara, CA, USA) .
2.4
Statistical methods
Three samples were photo-cured and analyzed for each sample geometry and beam profile except for sample geometry No. 1 and turbo light guide configuration where two samples were studied. Two-way ANOVA and Protected Least Significant Difference (PLSD) Fisher’s tests were applied to evaluate statistical differences in the data collected under different experimental conditions.
3
Results
The irradiance beam profiles of the Sapphire® LCU with the three light guide configurations and Bluephase Style LCU are presented in Fig. 1 . Fig. 1 (a) shows that the beam profile for the PAC unit with the standard light guide is uniform over the central 8 mm diameter region with a mean irradiance of 1.04 W/cm 2 and peak to peak fluctuation of 0.18 W/cm 2 . The full width at half maximum (FWHM) which characterizes the beam spatial extent is 9.5 mm; comparable to the 9 mm sample diameter. Fig. 1 (b) depicts the irradiance beam profile of the Sapphire® LCU with a turbo tip light guide and is described by a bell-like shape with a maximum irradiance of 15 W/cm 2 and a FWHM of 2 mm; the latter is five times smaller than the RBC sample diameter. The irradiance beam profile of the Sapphire® LCU obtained with a standard light guide and a 250 μm diameter aperture attachment placed on the light guide tip is depicted in Fig. 1 (c). This LCU configuration exhibits a narrow beam profile with a peak irradiance of 85 mW/cm 2 and a FWHM of 0.5 mm which is 20 times smaller than the sample diameter. In Fig. 1 (d), the irradiance beam profile of the Bluephase Style LCU was acquired at 0 mm distance from the light guide tip. The profile consists of three high irradiance regions 2.5 mm diameter each and separated by 4 mm corresponding to the three LEDs in the LCU head. Maximum irradiances of 6 W/cm 2 and 2 W/cm 2 are associated with each of the two 460 nm and one 405 nm emission wavelength LEDs, respectively.
Fig. 2 depicts the 2D shrinkage field at four representative times for the Filtek Supreme Ultra CT in the bonded disk geometry No. 1 and for the standard, turbo, and narrow beam profiles. The LCU exposure time was 30 s for the standard and turbo beam profiles while it was 720 s for the very low irradiance narrow beam profile. For the standard and turbo beam profiles, the deflection and deflection rate measured at the center of the 2D shrinkage field vary with time in the same way as with past observations . In addition, the 2D shrinkage fields for the three beam profiles are not flat, but take on distinct concave shapes with time. For the standard beam profile, which has the highest irradiance uniformity among all beam profiles, the 2D shrinkage field evolves from a concave surface with a small 2–3 μm depression at its center at 0.97 s to a more pronounced concave surface at 2.03 s. At the last two times shown, the 2D shrinkage field takes a bowl shape with a central 4 mm diameter flat bottom. For the turbo beam profile, the 2D shrinkage field evolves into a conical shape at short times up to about 5 s, and then a dimple like region develops with time resulting in a M-like shape near the center at long times. For the narrow beam profile, the 2D shrinkage field initially evolves in a conical manner as for the turbo beam profile, however, a protrusion develops at the center of the field at long times.
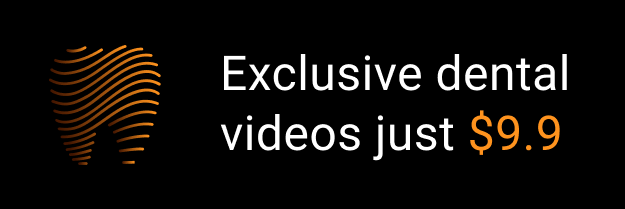