24
Regenerative Medicine in Orthodontic Therapy
Nina Kaukua1, Kaj Fried2, and Jeremy J. Mao3
1 Tandreguleringscenter Fyn, Odense, Denmark
2 Department of Neuroscience, Karolinska Institutet, Stockholm, Sweden
3 Center for Craniofacial Regeneration, Columbia University, New York, NY, USA
Orthodontics and dentofacial orthopedics (ODO) form “the branch of dentistry that specializes in the diagnosis, prevention and treatment of dental and facial irregularities. The practice of orthodontics requires professional skills in the design, application and control of corrective appliances to bring teeth, lips and jaws into proper alignment and to achieve facial balance” (American Association of Orthodontists, https://aaoinfo.org). Contemporary orthodontics is widely considered to have originated in the early years of the twentieth century, when Edward Hartley Angle began to practice fixed appliance treatment, by dragging and/or pushing malaligned teeth into esthetic positions with metallic bands and brackets cemented to the teeth. Over the past century, orthodontics, or the field later named orthodontics and dentofacial orthopedics, has witnessed few fundamental innovations, although many diagnostic and therapeutic refinements have been instituted (Mao et al., 2010).
Regenerative medicine is defined as the process of replacing or “regenerating” human cells, tissues, or organs to restore or establish normal function. This field holds the promise of regenerating damaged tissues and organs in the body by replacing damaged tissue or by stimulating the body’s own repair mechanisms to heal tissues or organs. Regenerative medicine also enables scientists to grow tissues and organs in the laboratory and safely implant them when the body is unable to heal itself. Orthodontics and regenerative medicine are two separate fields of study, and yet with a multitude of unrealized common threads. Both ODO and tissue engineering strive to improve tissue functions. Regenerative medicine is a much younger field in comparison with orthodontics, but has nonetheless witnessed robust growth in the fields of tissue healing and organ defects that are currently deemed incurable by contemporary medicine. The classic regenerative medicine paradigm involved the combination of living cells with a natural, synthetic, or bioartificial support to develop a biological substitute or a three‐dimensional (3D) living construct that is structurally, mechanically, and functionally equal to a tissue. Currently, researchers have gained high expertise in cell manipulation, materials science, and bioengineering for the design of highly complex biomimetic tissue substitutes for reparative/regenerative purposes, leading to the development of 3D engineered models designed to recapitulate the particular conditions that are intended to be mimicked. (Figure 24.1; Caddeo et al., 2017). ODO is considered to be at a pivotal point of transformation, with digital technology–assisted treatment planning and execution, more incorporation of skeletal anchorage systems in treatment, and conducting more and more research on regenerative medicine methods. This chapter attempts to identify potential interactions between ODO and regenerative medicine, including situations in which ODO may generate translational and clinical motivations for tissue engineering.

Figure 24.1 (a) The classic tissue engineering paradigm consists in the isolation of primary cells from the patient and further seeding on a three‐dimensional (3D) porous scaffold, specifically designed to induce cell proliferation and differentiation. The bioartificial system is developed in a specific environment, where the metabolic, mechanical, and electrical stimuli are provided by a bioreactor. In this tissue‐specific designed system, cells start to produce extracellular matrix, leading to in vitro tissue maturation. The tissue‐engineered construct is then implanted in the patient, undergoing to a remodeling process in vivo, which allows tissue restoration. (b) The tissue engineering approach for the development of in vitro models. The first step is to understand what part of the system considered should be represented and which specific conditions should be met. The second step is the design of the appropriate scaffold, i.e. the design of a 3D porous construct recapitulating the architecture and the mechanical and surface properties of the tissue/organ to be modeled. The third step is the selection of the cells to be seeded in the designed scaffold. The subsequent steps should be the design and fabrication of a bioreactor and the selection of the appropriate chemical signals (e.g. cocktail of growth factors) to be included in the model. Once a model is successfully developed, it can be improved by increasing its complexity, by introducing an additional cell type or chemical factor, or coupling different mechanical stimuli. The final step is validation, aiming to demonstrate the relevance of the model for the intended purpose. The validation procedure is fundamental and this step should be carefully designed.
Source: Caddeo et al. (2017) / from Frontiers Media S.A., CC BY 4.0.
Principles of tissue regeneration
Tissue engineering is the construction of bioartificial tissues in vitro and/or the in vivo alteration of cell growth and function via implantation of suitable cells isolated from donor tissue and biocompatible scaffold materials. The basic tenets of tissue engineering have been to incorporate cells and scaffold and signaling molecules into in vitro analogs of tissues that are to functionally replace diseased or lost tissues (Figure 24.2; Langer and Vacanti, 1993; Rahaman and Mao, 2005; Scheller et al., 2009; Caddeo et al., 2017). Combinations of cells and materials can reorganize themselves based on the strength of adhesion between cells and substrate and among the various cell types present in the tissue construct. It is essential for the biomaterials used for tissue engineering to have controlled surface chemistry, porosity, and biodegradability so that optimal cell adhesion, migration, and deposition of endogenous extracellular matrix materials by the cells are promoted. The tissue constructs created should be able to intimately integrate into the host’s vascular system in order to provide efficient nutrient supply and waste removal. For tissue engineering to be successful, one must understand the structure and function of the tissue to be regenerated, and then apply this knowledge in the design of tissue engineering strategies.
Many challenges remain for tissue engineering of dental, oral, and craniofacial structures. Considerations such as high demand for esthetics, appropriate vascularization, the complex environment, and the need for accommodating multiple tissue phenotypes should be taken into account when dental, oral, and craniofacial tissues are candidates for tissue engineering. A number of approaches have been explored to determine and accommodate the need for regenerating craniofacial tissues.
Stem cell basics
In postnatal life, stem cells are generally those rare cells with the potential to differentiate into multiple cell types. Usually, stem cells are quiescent in the body and serve as reserve cells. Under physiological conditions, stem cells periodically replenish themselves in a process known as cycling or self‐renewal, to maintain a renewable stem cell pool (Watt and Hogan, 2000; Verfaillie, 2002). Self‐renewal is fundamental for biological tissues, not only to replace dead or aged cells, but also to repair pathological defects or trauma (National Institutes of Health, 2009). With the great deal of interest in the regeneration of dental, oral, and craniofacial tissues, one should not overlook the role of stem cells in maintaining tissue homeostasis. Tissue turnover is exemplified most obviously by epithelial cells of the skin and by bone cells. Skin and bone, as well as many other tissues, undergo physiologically necessary turnover throughout life.
Stem cell characteristics
Stem cells are unspecialized cells and are important tools owing to their capacity to differentiate into a large number of cells according to the stimuli provided. These require the correct medium containing the factor that will provide the desired stimulation to guided differentiation along the macroenvironment with adequate temperature, pH, gas rate (such as a bioreactor), and a 3D structure to provide the right microenvironment for cell proliferation, growth, and differentiation (scaffold; Tonelli et al., 2017). For example, mesenchymal stem cells are capable of differentiating into cells such as osteoblasts, fibroblasts, chondrocytes, and myocytes (Figure 24.3) and can give rise to bone, cartilage, bone marrow stroma, interstitial fibrous tissue, skeletal muscle, tendons, ligaments, as well as adipose tissue (Jiang et al., 2002; Mezey et al., 2003; Alhadlaq et al., 2005; Sonoyama et al., 2005; Marion and Mao, 2006; Stosich and Mao, 2007; Lee et al., 2010a). Stem cells can be classified as follows (Zakrzewski et al., 2019):

Figure 24.2 Traditional application of tissue engineering. Appropriate cells such as (a) stem cells, (b) bioactive molecules, and (c) a compatible scaffold are mixed together for the correct tissue formation of the craniofacial area, such as teeth, periodontium, temporomandibular joints, and calvarial bone.

Figure 24.3 (a, b) Mesenchymal stem cells (MSCs). (c) MSCs subjected to osteogenic stimulation readily differentiated into osteogenic cells that elaborated minerals. (d, e) Safranin O staining is negative in connective tissue growth factor (CTGF)‐treated MSCs (e), as in MSCs without CTGF treatment (d). (f) In contrast, MSCs subjected to chondrogenic stimulation readily differentiated into Safranin O positive chondrogenic cells. MSC‐Fb, MSC differentiated into fibroblasts. Scale bars: 100 μm.
Source: Lee et al. (2010) / courtesy of the American Society for Clinical Investigation.
- Totipotent stem cells. Totipotency has the highest differentiation potential and allows cells to form both embryonic and extraembryonic structures. The zygote, which is formed after a sperm fertilizes an egg, is the classic example of a totipotent stem cell. These stem cells can later either develop into any of the three germ layers or form a placenta. After approximately four days, the blastocyst’s inner cell mass is the source of pluripotent cells called embryonic stem cells (ESCs).
- Pluripotent stem cells (PSCs) form cells of all germ layers, but not extraembryonic structures such as the placenta. ESCs derived from the inner cell mass of preimplantation embryos are a typical example. Another example is induced pluripotent stem cells (iPSCs) derived from the epiblast layer of implanted embryos. Their pluripotency is a continuum, starting from completely pluripotent cells such as ESCs and iPSCs and ending as representatives with less potency: multi‐, oligo‐, or unipotent cells. Their culturing and utilization seem to be very promising in regenerative medicine.
- Multipotent stem cells have a narrower spectrum of differentiation than PSCs, but they can specialize in discrete cells of specific cell lineages. One example is a hematopoietic stem cell, which can develop into several types of blood cells. After differentiation, a hematopoietic stem cell becomes an oligopotent cell. Its differentiation abilities are then restricted to cells of its lineage.
- Oligopotent stem cells can differentiate into several cell types. A myeloid stem cell is an example that can divide into white blood cells but not red blood cells.
- Unipotent stem cells are characterized by the narrowest differentiation capabilities and a special property of dividing repeatedly. This latter feature makes them a promising candidate for therapeutic use in regenerative medicine. These cells are only able to form one cell type.
A detailed description of ESCs (totipotent and pluripotent), adult stem cells (multipotent, oligopotent, and unipotent), and iPSCs follows.
Embryonic stem cells
ESCs are found in the inner cell mass of the human blastocyst. In normal embryonic development, they disappear after the seventh day in order to form the three embryonic tissue layers. The blastocysts formed after the fusion of sperm and ovum fertilization are composed of two distinct cell types: the inner cell mass (ICM), which develops into epiblasts and induces the development of a fetus, and the trophectoderm. As the trophectoderm begins to form a specialized support structure, such as the placenta, the ICM cells remain undifferentiated, fully pluripotent, and proliferative, allowing them to form any cell of the organism. Human embryonic stem cells (hESCs), derived from the ICM, form aggregations called germ layers during the process of embryogenesis to endoderm, ectoderm, and mesoderm (Figure 24.4). After ESCs differentiate into one of the germ layers, they become multipotent stem cells, whose potency is limited to only the cells of the germ layer. After that, PSCs occur all over the organism as undifferentiated cells, and their key abilities are proliferation by the formation of the next generation of stem cells and differentiation into specialized cells under certain physiological conditions.
In 1998 hESCs were successfully grown in the laboratory for the first time (Thomson et al., 1998). Undifferentiated ESCs extracted from the inner cell mass during the blastocyst stage can be cultured in the laboratory and under the right conditions will proliferate indefinitely and retain the potential to differentiate into cells of all three embryonic tissue layers. Research involving hESCs is at the center of the ethical debate about stem cell use and potential in regenerative medicine (National Research Council (US) and Institute of Medicine (US) Committee on the Biological and Biomedical Applications of Stem Cell Research, 2002).
Adult stem cells
Somatic or adult stem cells are undifferentiated and found among differentiated cells in the whole body after development. The adult stem cells have the capacity to self‐renew themselves into a limited number of mature cell types and help in maintaining tissue homeostasis. They are usually maintained in a quiescent state, but on the loss of cells or injury to the tissue they can be activated to proliferate and differentiate into the required type of cells (Watt and Hogan, 2000; National Institutes of Health, 2009). Among many types, there are the following (Zakrzewski et al., 2019):
- Mesenchymal stem cells (MSCs) are present in many tissues. In bone marrow, these cells differentiate mainly into bone, cartilage, and fat cells. As stem cells, they are an exception because they act pluripotently and can specialize in the cells of any germ layer.
- Neural cells give rise to nerve cells and their supporting cells: oligodendrocytes and astrocytes.
- Hematopoietic stem cells (HSCs) form all kinds of blood cells: red, white, and platelets.
- Skin stem cells form, for example, keratinocytes, which create a protective layer of skin.
Bone marrow is the source of at least three stem cell populations: MSCs, HSCs, and proangiogenic progenitor cells (CD34+ and CD133+). Isolation of MSCs from bone marrow was first described by Friedenstein and collaborators (1974). MSCs isolated by adherence to culture plates are heterogeneous. Only a fraction of cells among typical MSC preparations are stem cells (Lee et al., 2010b). Through employing suitable media and growth supplements that initiate lineage differentiation, human MSCs (hMSCs) have the capacity to differentiate into all the three lineages; that is, ectoderm (neuronal cells and dental‐derived MSCs are one source of generating these cells), mesoderm (adipocytes, osteocytes, and chondrocytes) and endoderm (hepatocytes and pancreocytes; Zakrzewski et al., 2019). Several studies have explored the potential of MSCs in the regeneration of craniofacial tissues, such as alveolar bone, periodontium, and even ectomesenchymal‐based tooth structures (Kawaguchi et al., 2004; Maria et al., 2007; Kim et al., 2010a; Kim et al., 2010b).

Figure 24.4 Oocyte development and formation of stem cells. The blastocoel, which is formed from oocytes, consists of embryonic stem cells that later differentiate into mesodermal, ectodermal, or endodermal cells. Blastocoel develops into the gastrula.
Source: Zakrzewski et al. (2019) / Springer Nature / CC BY‐4.0.
HSCs are important because they are by far the most thoroughly characterized tissue‐specific stem cell. HSCs are coinhabitants of bone marrow with MSCs, and MSCs and osteoblasts serve as stromal cells for HSCs. HSCs are responsible for the generation of all functional hematopoietic lineages in blood, including erythrocytes, leukocytes, and platelets. HSC transplantation solves problems that are caused by inappropriate functioning of the hematopoietic system, which includes diseases such as leukemia and anemia (Zakrzewski et al., 2019). The osteoclasts, the bone‐resorbing cells during bone turnover and orthodontic tooth movement, derive from this hematopoietic lineage (Kyba, 2005).
Induced pluripotent stem cells
iPSCs are adult somatic cells that have been genetically reprogrammed to an ESC‐like state through the forced expression of genes and factors important for maintaining the defining properties of ESCs. It was Yamanaka’s laboratory at Kyoto University that developed mouse iPSCs from mouse fibroblasts in 2006. Human iPSCs were first independently produced by Yamanaka’s and Thomson’s groups from human fibroblasts in late 2007. Human iPSCs have been successfully generated from adipocyte‐derived stem cells, amniocytes, peripheral blood, cord blood, dental pulp cells, oral mucosa, and skin fibroblasts. Various growth factors and chemical compounds have recently been found to improve the induction efficiency of iPSCs. Nakagawa et al. (2008) have shown that iPSCs can be generated from fibroblasts by viral integration of Oct4/Sox2/Klf4. This suggests that endogenous expression of transcription factors, which maintain stemness, have a role in the reprogramming process of pluripotency. Even though retroviruses are being extensively used to reprogram somatic cells into iPSCs, retroviral vectors may have significant risks that could limit their use in patients (Ye et al., 2013). In the current state, a better understanding of epigenetic alterations and transcriptional activity associated with the induction of pluripotency and following differentiation is required for the efficient generation of therapeutic cells. Long‐term safety data must be obtained to use human iPSC‐based cell therapy for the treatment of diseases.
Dental tissues as a source of stem cells
Cranial neural crest cells are essential for the development of craniofacial structures. The head is formed through an interaction between mesodermal and cranial neural crest cells. The neural crest arises from the ectoderm‐derived neural tube during prenatal development at approximately day 20 in the human embryo (Bhattacherjee et al., 2007). These cells migrate to the presumptive face, and possess the ability to self‐renew and differentiate into multiple cell types for the genesis of teeth, bone, and cartilage. How neural crest cells differentiate into craniofacial tissues is only partially understood. The cranial neural crest cells migrate into the pharyngeal arches, disperse throughout this region, and give rise to the ectomesenchyme, which will interact with its surroundings to form the diverse tissues already mentioned as well as other tissues such as the neurons and glia of cranial ganglia (Chai et al., 2000; Graham and Smith, 2001; Le Douarin et al., 2008). Craniofacial bones are further developed by intramembranous ossification or deposition of bone matrix by osteoblasts. Tooth morphogenesis occurs via a series of complex signaling interactions between the oral epithelium (dental lamina) and the underlying ectomesenchyme (Olsen et al., 2000; Tucker and Sharpe, 2004). In comparison, the bones of the rest of the body form by mesoderm‐derived mesenchymal cells, through endochondral ossification where there is a chondrogenic template, before bone matrix is laid down (Vaglia and Hall, 1999; Olsen et al., 2000; Noden and Trainor, 2005).
Neural crest cells differentiate into ectomesenchymal progenitor cells in the cap stage of the dental follicle. The dental follicle surrounds the dental papilla and dental epithelium, and gives rise to cementum, periodontal ligament, and alveolar bone. Specifically, interactions between Hertwig’s epithelial root sheath and dental follicle lead to the differentiation of cementoblasts, osteoblasts, and fibroblasts. Important regulators of alveolar bone development include Runx2, Dlx 5/6, and Msx1 (Saito et al., 2009; Fleischmannova et al., 2010).
On completion of tooth morphogenesis, stem cells have been identified in several dental and periodontal structures, including the dental pulp of both deciduous and permanent teeth, papillae, periodontal ligament, dental follicles, and dental‐derived epithelial stem cells (Gronthos et al., 2000; Miura et al., 2003; Seo et al., 2004; Honda et al., 2007; Sonoyama et al., 2008; Yao et al., 2008). It should come as no surprise when stem cells are discovered in any biological tissues, given the fundamental roles of stem cells to maintain tissue homeostasis and participate in tissue repair following trauma or pathological insults.
The various sources of dental progenitor cells include the dental pulp stem cells (DPSCs; Gronthos et al., 2000), stem cells from human exfoliated deciduous teeth (SHED; Miura et al., 2003), periodontal ligament stem cells (PDLSCs; Seo et al., 2004), dental follicle stem cells (DFSCs; Morsczeck et al., 2005), stem cells from apical papilla (SCAP; Sonoyama et al., 2008), and gingival stem cells (GING SCs; Mitrano et al., 2010). Like bone marrow–derived mesenchymal stem cells (BM‐MSCs), dental‐derived stem cells exhibit self‐renewal capacity, possess high proliferation rates, have multilineage differentiation potential into a wide spectrum of cell types from the three germ layers, or, at least in part, express their specific markers under the appropriate culture conditions. Despite being similar at a coarse level, the transcriptomic and proteomic profiles of oral stem cells reveal several molecular differences, including differential expression of surface marker, structural proteins, growth hormones, and metabolites, indicating prospective developmental divergence (Hosmani et al., 2020; Krivanek et al., 2020). All these characteristics suggest that dental stem cells might be the optimal choice for tissue self‐repair and regeneration (Al Madhoun et al., 2021).
Dental pulp stem cells
DPSCs, the most studied dental stem cells, are ectoderm‐derived stem cells originating from migrating neural crest cells. DPSCs were initially isolated and characterized by Gronthos et al. (2000). The culturing method and accurate characterization are pivotal steps for the isolation of high‐quality DPSCs. Following extraction of the teeth (especially and most appropriately a third molar), further procedures include mechanical extraction of the soft pulp connective tissue, maceration, enzymatic digestion of extracellular matrix (ECM) proteins, and cell growth in plastic tissue/cell culture plates. DPSCs remained viable up to five days after tooth storage at 4 °C in phosphate buffered saline (PBS), but viability was dramatically reduced after 24 hours of storage, indicating that teeth should be immediately processed to ensure the highest cell recovery (Perry et al., 2008). Ferro et al. (2014) recommended the use of fresh pasteurized milk for teeth transport due to its antimicrobial properties. Other procedures involve teeth or dental pulp storage with cryoprotectant agents (CPA; Rodas‐Junco and Villicaña, 2017). For DPSC isolation, the most widely used methods are enzymatic digestion (ED) of tissues or outgrowth (OG) from tissue explants. ED enables isolation of single‐cell suspensions from primary tissues by digestion with enzymes such as collagenase type I and II, dispase, trypsin, and accutase. On the other hand, the OG method is simpler and faster and consists of placing pulp fragments (1–2 mm3 pieces) directly into the culture plate, so that cells outgrow from the pulp tissue explants (Rodas‐Junco and Villicaña, 2017). Improving the optimal cell attachment in the plastic dish for primary culture involves precoating the plastic surfaces with ECM proteins, or using peptide‐modified surfaces, synthetic polymeric cations, or culture‐treated surfaces. The most common culture media used for DPSCs include alpha‐minimal essential medium (α‐MEM), Dulbecco’s modified Eagle medium (DMEM), DMEM/Ham’s F12 nutrient medium (DMEM/F12), DMEM low glucose (DMEM‐LG), and DMEM Knock Out (DMEM‐KO) media (Rodas‐Junco and Villicaña, 2017). Standard cell culture media are commonly supplemented with fetal bovine serum (FBS; also called fetal calf serum, FCS), which provides many components required for cell growth, metabolism, and proliferation. Basic fibroblast growth factor (bFGF, also known as FGF‐2) and epidermal growth factor (EGF) have been used routinely for MSC culture because they have a pronounced mitogenic effect and retain differentiation potential toward several lineages (Rodas‐Junco and Villicaña, 2017). A schematic representation of critical steps followed from tooth selection to DPSC cryopreservation and application is provided as Figure 24.5.
DPSCs are composed of progenitor cells that are marked by diverse characteristics, such as clonal heterogeneity, multilineage differentiation, self‐renewal capacity, and phenotypic complexity. DPSC populations are functionally heterogeneous, as evidenced through their surface antigen profile or expression patterns of a variety of markers that are associated with progenitors of different lineages. DPSCs show surface expression of STRO‐1, CD13, CD29, CD44, CD73, CD90, CD105, CD146, and CD166 – a profile that is reminiscent of BM‐MSCs. Additionally, DPSCs express various pluripotency markers, such as Oct‐3/4, Nanog, and Sox‐2 – that is, the stemness‐related markers observed in ESCs – which explains, at least in part, their self‐renewal potential (Faruqu et al., 2020). As neuronal crest–derived cells, DPSCs express several neural stem cell markers, including nestin, neuronal nuclei antigen, vimentin, synaptophysin, musashi‐1, Galactosyl‐ceramidase, S100 calcium binding protein B, neurofilament heavy (NFH) chain, class III β‐tubulin, and neurofilaments (Al Madhoun et al., 2021).
DPSCs could be a valuable source for cell therapy and advancing current regenerative medicine strategies. DPSCs have the potential to differentiate into endodermal (respiratory and gastrointestinal tracts, liver, pancreas, thyroid, prostate, and bladder lineages), mesodermal (adipogenic, osteogenic, and chondrogenic lineages), and ectodermal (skin and neural lineages; Yamada et al., 2019). In addition, DPSCs were shown to differentiate into myocytes, cardiomyocytes, hepatocyte‐like cells, melanocytes, and active neurons (Patil et al., 2014). DPSCs also have remarkable potential as alternative sources to multipotent MSCs and, due mainly to their immunomodulatory properties (mediating G0/G1 cell cycle arrest of the chemically activated T cells), DPSCs constitute a highly valuable source for cell therapy of a variety of inflammatory diseases and other disorders. With regard to basic or preclinical studies in experimental animal models, human DPSC‐based therapies have been successfully used in several disease conditions, including diabetes, neuropathy, hepatic diseases, oculopathies, spinal cord, peripheral nerve injury, cerebral ischemia, muscular dystrophy, myocardial infarction, Parkinson’s disease, lung injury, and stroke (Anitua et al., 2018; Al Madhoun et al., 2021).
Periodontal ligament stem cells
The periodontal ligament (PDL), the connective tissue that connects the root cementum and the alveolar bone, is derived from embryonic cranial neural crest cells (CNCCs). The periodontium includes bone and cementum cells and a mix of fibroblasts, mesenchymal, and undifferentiated cells, all of which are seated on a hydrated fibrillar extracellular substance rich in collagen. PDLSCs contain 95% MSCs and 5% neural crest stem cells (Ng et al., 2015). These cells have the ability to grow clonally and express MSC markers (vimentin, STRO‐1, CD90, scleraxis, and CD44), markers of ESCs (c‐Myc, SSEA4, Klf4, Oct4, Nanog, and Sox2), and neural crest cells markers (Sox10, nestin, Snail, Slug, Sox10 p75, and Tuj1; Coura et al., 2008; Song et al., 2012). These markers are considered as hallmarks of multipotency that highlight the differentiation capacity of PDLCs into various cell types, such as chondrogenic, cardiomyogenic, osteogenic, and neurogenic lineages.

Figure 24.5 Schematic representation of critical steps followed from tooth selection to dental pulp stem cell (DPSC) cryopreservation and applications. Each step summarizes critical factors that should be considered for optimization in order to improve DPSC culture and stability during manufacturing. For tooth selection, several factors related to donor characteristics must be considered to establish specific criteria according to research interests. As we can see, many key factors fall into cell culture formulation, suggesting that this issue is one of the most important to establish general or tailored protocols and obtain DPSCs with specific properties, as well as the long‐term maintenance of cultures. Homogeneous populations of DPSCs may be cryopreserved or directly applied to basic or clinical research, with either previous or simultaneous molecular and biochemical characterization. CPA, cryoprotective agents; FBS, fetal bovine system; HLA, human leukocyte antigen; HS, hematopoietic stem cells.
Source: Reproduced with permission from Rodas‐Junco and Villicaña (2017) / Springer Nature.
PDLSC isolation was described for the first time by Seo et al. (2004). PDLSCs can be easily harvested from periodontal tissue during the noninvasive procedure. The first and second premolar teeth are suitable for the purpose of PDLSC isolation. Teeth are transferred in the cell culture medium supplemented with 1% antibiotic at 4 °C. In all seeding techniques, complete DMEM (supplemented with L‐glutamine, 1% antibiotic, 10% FBS) is used in standard culture conditions (37 °C with 5% CO2). The cells are isolated with two different methods through enzymatic digestion with type 1 collagenase or trypsin/EDTA and mechanical disaggregation and explant culture. Since PDLSCs have the same embryological origin of the neural crest and are less lineage divergent than neural cells, they are considered promising for neuroregenerative applications. Additionally, PDLSCs have an immunomodulatory ability that is comparable to BM‐MSCs (Zhu and Liang, 2015). Briefly, PDLSCs can differentiate into cementoblasts, osteoblasts, and fibroblasts, the main cell types responsible for guaranteeing tissue homeostasis of the cementum, alveolar bone, and PDL, respectively, which highlights their application in regenerative periodontology. PDLSCs can also differentiate into neurons, cardiomyocytes, endothelial cells, pancreatic islet cells, and corneal keratocytes, which further expands their application in regenerative medicine. Eventually, PDLSCs have immunomodulatory properties that can be performed by direct cell‐to‐cell contact or by the synthesis of specific metabolites that alter the phenotype of different immune cells. Despite some promising clinical results and biological insights, several gaps need to be filled before adopting a protocol for their clinical use in patients with periodontitis or other inflammatory conditions (Trubiani et al., 2019; Queiroz et al., 2021).
Gingival mesenchymal stem cells
The human gingiva is a unique masticatory keratinized mucosal tissue and an essential component of the periodontal apparatus, the connective tissue of which develops from both the neural crest and the mesenchyme. The initial steps toward isolation and characterization of gingival MSCs (GMSCs) were made by Zhang et al. (2009). Accumulating studies have demonstrated the promising potential of GMSCs as a readily accessible and expandable source of MSCs that can be easily obtained through minimally invasive surgical techniques (Górski, 2016; Fawzy El‐Sayed and Dörfer, 2016). Initially, it was found that human gingival tissues harbor clustered immunostaining signals for stem cell–related genes such as Oct‐4, SSEA‐4, and STRO‐1 in the lamina propria/connective tissue layers, suggesting the existence of a subpopulation of adult stem cells in human gingival tissues. Subsequently, a unique population of progenitor cells was isolated from normal human gingival tissues, which are characterized by their fibroblast‐like spindle morphology, the colony‐forming unit–fibroblast (CFU‐F), the expression of a panel of MSC‐related cell surface markers such as CD73, CD90, CD105, SSEA‐4, and STRO‐1 but negative for hematopoietic cell markers like CD34 and CD45, and multipotent differentiation into osteocytes, adipocytes, and neural types of cells. The properties of these progenitor cells derived from human gingival tissues fulfill the minimal criteria of the International Society for Cell & Gene Therapy (ISCT) to define mesenchymal stromal/stem cells, thus designated gingiva‐derived mesenchymal stem/stromal cells (Kim et al., 2021). In terms of their multipotency, GMSCs not only possess mesodermal trilineage differentiation potentials (osteocytes, adipocytes, and chondrocytes), but also the potential to transdifferentiate into ectodermal and endodermal cell lineages, such as neural cells, keratinocytes, endothelial cells, and odontogenic cells, under defined induction conditions. Easy accessibility, less morbidity of harvesting, the highly proliferative activity and genomic stability, and a neural crest origin as well as the potent immunomodulatory and regenerative potentials make GMSCs an attractive source of adult stem cells for tissue engineering and regenerative therapy (Kim et al., 2021).
Three‐dimensional scaffolds
The purpose of fabricating scaffolds is to produce tissue‐like materials such that they can eventually perform like the native tissues. The idea of scaffold fabrication is based on creating materials with optimum pore size, structure, and porosity for various applications. Generally, scaffolds are created first and cells are subsequently cultured on these scaffolds. Porous 3D scaffolds can be fabricated from various conventional and rapid prototyping techniques, depending on the type of materials used or type of pore structures needed. Open porous and interconnected networks are essential for cell nutrition, proliferation, and migration for tissue vascularization and formation of new tissues. Materials with high porosity enable effective release of biofactors such as proteins, genes, or cells, and provide good substrates for nutrient exchange. However, the mechanical property that is important in maintaining the structural stability of the biomaterial is often compromised as the result of increased porosity. These scaffolds serve to provide suitable microenvironments to support cell growth and function.
Various conventional techniques such as salt leaching, gas forming, phase separation, and freeze drying (Figure 24.6), as well as rapid prototyping techniques using computer‐aided design (CAD) modeling, are utilized for fabrication of scaffolds (Figure 24.7). Fabrication using conventional methods does not enable precise control of internal scaffold architecture or fabrication of complex architectures. Moreover, scaffolds fabricated using these traditional processing techniques have compressive moduli at a maximum of 0.4 MPa, which is much lower than hard tissue (10–1500 MPa) or most soft tissues (0.4–350 MPa). Such fabrication also uses toxic solvents, which may result in cell death if they are not completely removed. Rapid prototyping fabrication techniques enable the fabrication of scaffolds with improved mechanical properties, with moduli ranging from soft to hard tissues, with better design repeatability, part consistency, and control of scaffold architecture at both micro and macro levels. The main restriction is the limited number of biomaterials that can be processed by rapid prototyping compared to conventional techniques. Other approaches that mimic the way in which tissues are formed in the body, and their nanofibrous structure, can be found in modular assembly methods and electrospinning methods (Loh and Choong, 2013). A summary of scaffolds used for various tissue regeneration experiments is provided in Table 24.1.

Figure 24.6 Schematic drawing of the biopolymer‐forming process of conventional techniques for scaffold fabrication.
Source: Khalil et al. (2020) / MDPI / CC BY‐4.0.

Figure 24.7 Schematic drawing of biopolymer‐forming rapid prototyping techniques for scaffolds.
Source: Khalil et al. (2020) / MDPI / CC BY‐4.0.
Table 24.1 Summary of the biomaterial class, scaffold, porosity range, and target tissue.
Source: Adapted from Mallick and Cox (2013).
Material class | Scaffold composition | Fabrication type | Pore size/porosity (μm/%) | Target tissue |
---|---|---|---|---|
Ceramic |
HAP |
Sintering | 90–600 | Bone |
SFF and image based | 366, 444/38, 44 | Bone | ||
Porous sintering | 200–800/60–80 | Bone | ||
Sintering (solid, porous particles, and porous coral replication) | 50, 230/66, 70 | Bone | ||
TCP cement | Salt leaching | 0.2, 8.7/31, 62 | Bone | |
Calcium metaphosphate | Porous sintering | 200 | Bone | |
Natural coral | Porous sintering | 150–220/36 | Bone | |
HAP‐TCP | Porous cylinder sintering | 100–150/36 | Bone | |
Calcium phosphate | Compaction | – | Drug delivery | |
Glass and ceramic particles |
Porous glassy carbon | Porous pellet sintering | 100–200/40 | Bone |
Na2O–CaO–SiO2–P2O5 | Reaction sintering under isostatic pressure | 100–200 | Bone | |
Bioglass 45S5 | Melt derived | – | Bone | |
Foaming and porous sintering | 100–600 | Bone | ||
SiO2‐based sol‐gel foams | Foaming | <10–500 | Bone | |
Silica‐calcium phosphate | Phase transformation | 10–300/43–51 | Bone | |
Hyaluronic acid | Phase inversion, particulate leaching | 100–600/80–90 | Bone | |
Mesoporous bioactive glass | Polyurethane sponge technique Immersion in phosphate‐buffered saline solution | 0.002–0.05 | Drug delivery | |
Natural polymers | Collagen | Freeze drying | 11–134 | Bone |
Collagen/hyaluronate | Cross‐linking | 45.7, 35.4 | Bone | |
Silk fibroin | Freeze drying, salt‐leaching, gas foaming | 15–100/84–99 | – | |
Salt leaching | 202/84–98 | Bone | ||
Chemically synthesized polymers |
PLA | Phase separation, emulsion‐ solvent diffusion, and porogen leaching | 50–800 | Bone |
PLA/PGA | Melt processing | 800 | Bone | |
PLDLA | Solution coating, porogen decomposition | 58–80 | Bone | |
PLGA |
Sintered microsphere method | 83–300/35 | Bone | |
Consolidation by pressure drop | 100/60–66 | Teeth implants | ||
Sintering | 187/31 | Bone | ||
Gas foaming | 200 | Bone | ||
Electrospinning | 2–465/92 | – | ||
PLGA/PEG | Porogen dissolving | 300–500/85 | Articular cartilage | |
PLGA/PVA | Melt molding particulate leaching method | 200–300/90 | Bone | |
Poly (multifunctional lactic acid‐based oligomer) | Salt leaching | 45–150, 300–600/80 | Bone | |
PPF | Gas forming with effervescent reaction | 50–1000/51 | Bone | |
Porogen leaching and photo cross‐linking | 300–800/57–80 | Bone | ||
Gas forming with effervescent reaction | 100–500 | Bone | ||
PET | Melt blowing | 93–97 | Bone | |
Polymeric foams | High internal emulsion phase | 40, 100/70–97 | Bone | |
Poly(desaminotyrosl‐tyrosine ethyl ester carbonate) | Solid freeform | 486/80–87.5 | Bone | |
Composites |
PLLA/collagen/chitosan | Solvent castings Salt leaching |
125–500 | Bone |
PLGA/bioactive microsphere glass | Microsphere heating mold | 350–500 | Bone | |
PPF/PLGA‐PEG microparticles | Salt leaching | – | Bone | |
PLA/calcium metaphosphate | Porous sintering | 100–400 | Bone | |
PLLA/nHAP/collagen/chitin fibres | Ultrasonication, lyophilized | 200 | Bone | |
PLGA/HAP | Gas forming Particulate leaching |
100–250 | Bone | |
L‐PLGA/TCP D, L‐PLGA/L‐PLA | TheriForm 3D printing | 40–150/55, 90 | Articular cartilage | |
PGA fiber/fibrin | Freeze drying | 300 | Skin | |
PLGA mesh/collagen sponge gel | Knitted mesh | – | Urinary bladder | |
PLLA braid/collagen coating | Freeze drying | 50–100 | Ligament | |
PCL fiber/pHEMA hydrogel | Polymerization, acetone etched | 100–400/34–41 | Neural tissue engineering | |
PLGA/chitin | Electrospinning | – | Skin | |
PGA mesh/bioglass 45S5 | Bioglass particle in distilled water immerses PGA mesh |
– | Soft tissue | |
PLGA/bioglass tubular foam | Dispersion Freeze drying |
10–50, 100 | Intestine, trachea, blood vessel | |
PLA/nHAP/collagen | Phase separation | – | Periodontal tissues | |
HAP/PCL | Polymeric reticulate method | 150–200/87 | Drug delivery | |
HAP/chitosan‐gelatin | Freeze drying | 300–500/91 | Bone | |
HAP/beta‐TCP/chitosan | Porogen leaching Freeze drying |
300–600 | Bone | |
Collagen/HAP | Freeze drying | 30–100/85 | Bone | |
CO3Ap/collagen | Freeze drying | 50–300/49, 73, 79 | Bone | |
Titanium alloy (Ti‐6Al‐4V)/CaP sol‐gel | Porous sintering | 50–200/35 | Bone | |
Sintering (porous) | 250/86 | Bone | ||
Titanium/nHAP | Soaking | – | Bone | |
Ti‐TiBx, Ni‐Ti | Self‐propagating high‐temperature synthesis (porous) | 15–55 | Bone | |
PPF/beta‐TCP | Salt leaching | 150–300/69, 74 | Bone | |
PLLA/bioglass | Phase separation | <10, >100 | Bone | |
Silica‐calium phosphate | Sintering | 10–300/43–51 | Bone | |
PLGA/collagen/apatite | Salt leaching | 355–425/87 | Bone | |
Beta‐TCP/calcium phosphate invert glass/chitosan | Thermally induced phase separation, immersion in phosphate‐buffered saline solution | – | Drug delivery | |
Bioactive glass/beta‐cyclodextrin | Sol‐gel | – | Drug delivery | |
Metals |
Ni‐Ti | Self‐propagating high‐temperature synthesis | 229–641/47–66 | Bone |
Titanium fiber meshes | Sintering | 250/86 | Bone | |
Titanium |
Plasma spraying | 200–400/50–60 | Bone | |
Porous sintering | 50–200/35 | Dental | ||
Diffusion | 350/45 | Bone | ||
Laser texture (Ti‐6Al‐4V) | 100, 300 | Bone | ||
Electrochemical oxidation | 1–8/13–24 | Bone | ||
Shot blasting | <10/44, 48 | Bone | ||
Acid etching | 400 | Bone | ||
Deposition through polystyrene latex beads | 0.5, 16, 50 | Bone |
3D, three‐dimensional; CaP, calcium phosphate; CO3Ap, carbonate apatite; HAP, hydroxy apatite; L‐PLA, L‐poly(lactic acid); L‐PLGA, L‐ poly D,L‐lactic‐co‐glycolic acid; nHAP, nano‐hydroxy apatite; Ni‐Ti, nickel–titanium; PCL, poly(ε‐caprolactone); PEG, polyethylene glycol; PET, polyethylene terephthalate; PGA, polyglycolic acid; pHEMA, poly(2‐hydroxyethylmethacrylate); PLA, poly(lactic acid); PLDLA, poly‐L‐co‐D,L‐lactic acid; PLGA, poly(D,L‐lactide‐co‐glycolide); PLLA, poly‐l‐lactic acid; PPF, poly(propylene fumarate; PVA, polyvinyl alcohol; SFF, solid free form; TCP, tissue culture polystyrene; TCP D; Ti‐6Al‐4V, titanium, 6 aluminium, 4 vanadium; Ti‐TiB, titanium‐titanium boride.
Biomaterials
Biomaterials are frequently, but not always, needed for tissue engineering and tissue regeneration. For example, stem cells are infused for the repair of cardiac infarcts and can be delivered into cardiac muscle defects, typically without biomaterials as scaffolds. In contrast, a scaffold is frequently required for the regeneration of most dental, oral, and craniofacial structures, because they are 3D structures of functional and esthetic importance. A variety of scaffolds have been developed as extracellular matrix analogs, capable of supporting cells. At a minimum, biomaterial scaffolds should be biocompatible, nontoxic, and promote tissue regeneration (Box 24.1). Biodegradable materials are frequently desirable, given their predesigned purpose to undergo controlled degradation while tissue genesis takes place (Scheller et al., 2009).
Biomaterials can be of native origin, synthetic, or hybrids. The natural polymers, such as collagen, have the advantage of biocompatibility, whereas synthetic polymers allow precise control of physicochemical properties such as mechanical properties, degradation rate, porosity, and microstructures (Sharma and Elisseeff, 2004). Some of the popular synthetic biomaterials include co‐polymers of polylactic‐glycolic acid collagen, polyphosphazenes, polyurethanes, polycaprolactone, polyethylene glycol (PEG), poly(propylene fumarate), starch‐based materials, alginate, silk, bioactive glasses and glass ceramics, calcium‐phosphate ceramics, calcium‐phosphate and collagen blends, and synthetic polymer/apatite composites (Scheller et al., 2009).

VIDEdental - Online dental courses
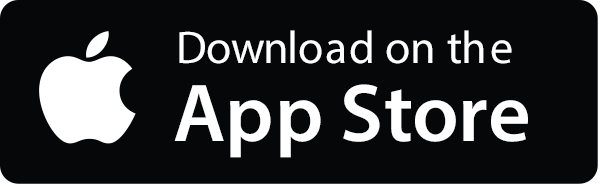
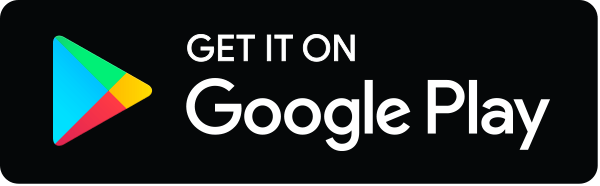