Abstract
The key elements for bioartificial bone formation in 3D matrices are large numbers of osteogenic cells and supplies of oxygen and nutrition. Vascularization becomes more important with the increasing size and complexity of seeded scaffolds required for clinical application in reconstructive craniomaxillofacial surgery. Prefabrication of vascularized bioartificial bone grafts in vivo might be an alternative to in vitro tissue engineering techniques. Two cylindrical β-TCP-scaffolds (25 mm long) were intraoperatively filled with autogenous bone marrow from the iliac crest for cell loading and implanted into the latissimus dorsi muscle in 12 sheep. To determine the effect of axial perfusion, one scaffold in each sheep was surgically supplied with a central vascular bundle. Sheep were killed 3 months after surgery. Histomorphometric analysis showed autogenous bone marrow from the iliac crest was an effective source of osteogenic cells and growth factors, inducing considerable ectopic bone growth in all implanted scaffolds. Bone growth, ceramic resorption and angiogenesis increased significantly with axial perfusion. The results encourage the application of prefabricated bioartificial bone for segmental mandibular reconstruction in man. In clinical practice, vascularized bioartificial bone grafts could change the principles of bone transplantation with minimal donor site morbidity and no shape or volume limitations.
Osseous defects resulting from tumor resection, trauma or infection remain a challenging problem in reconstructive craniomaxillofacial surgery . Autogenous bone grafts represent the gold standard and are frequently used clinically for osseus reconstruction . After transplantation, the cells and matrix compounds of autogenous bone grafts provide high osteogenic potential, which leads to complete substitution by newly formed bone within a gradual remodeling process. In situations with compromised perfusion due to radiation or infection, microsurgical bone transfer is preferred for osseous reconstruction, inducing a healing process from the transplanted graft comparable to bone fracture healing . Bone harvesting is associated with considerable donor site morbidity, which increases with the amount of harvested bone . The amount of autogenous bone for transplantation is limited and the volume and shape of autogenous bone grafts may compromise prosthetic rehabilitation especially after microsurgical bone transfer . Processed allogenic and xenogenic bone grafts are alternatives but are used with reservation because of the risk of disease transmission. Higher complication rates after allogenic and xenogenic bone transplantation indicate a lower osteogenic potential compared with autografts, even when osteoinductive factors are preserved during processing .
The generation of bioartificial bone grafts using tissue engineering techniques is an attempt to overcome the limitations of the methods mentioned above. Until now, tissue engineering techniques with cultivation of osteogenic cells and seeding on scaffolds in vitro did not seem effective enough to generate large bioartificial bone grafts for clinical use . The key elements for bioartificial bone formation in three-dimensional (3D) matrices in any tissue engineering concept are a high number of osteogenic cells and supplies of oxygen and nutrition. Vascularization becomes more important with the increasing size and complexity of the seeded scaffolds required for clinical application in reconstructive craniomaxillofacial surgery .
Various vascularization concepts for 3D matrices are the focus of experimental research . Combining tissue engineering approaches with flap prefabrication techniques may allow the application of vascularized bioartificial bone grafts grown in vivo with the advantage of minimal donor site morbidity compared with conventional bone grafts. In this study, osteogenic material from the iliac crest was obtained intraoperatively and used directly for cell loading 3D matrices in a surgical seeding procedure. The latissimus dorsi muscle served as a natural bioreactor to overcome the limitations of extracorporal tissue engineering techniques with cell expansion in vitro . The goal of this study was to determine the effect of this seeding procedure on bone formation within large bioartificial scaffolds, especially in combination with axial perfusion and whether this technique leads to sufficient bone growth for clinical use.
Materials and methods
Cylinders of 25 mm length and 14 mm diameter consisting of pure β-tricalcium phosphate with a porosity of 60–80% and a pore size of 100–500 μm were used as carriers (ChronOs ® , Synthes, West Chester, USA). The cylinders were modified with a central passage of 7 mm diameter and sterilized again by gamma radiation. Animal experiments were conducted under a protocol approved by the ethics committee in accordance with German federal animal welfare legislation. Twelve healthy adult female German black-headed sheep with an average weight of 72.5 ± 7.4 kg were included in the study. After intravenous induction (1 ml midazolam, 5 mg/kg propofol) anesthesia was maintained with isoflurane delivered in 100% oxygen (1 l/min). Additionally, buprenorphine (10 μg/kg i.m.), carprofen (4 mg/kg half i.v., half s.c.) and fentanyl (0.005 mg/kg i.v.) were applied for analgesia. Systolic, diastolic and mean blood pressure, electrocardiogram, rectal temperature and hemoglobin oxygen saturation were continuously monitored during surgery. Three bone biopsies (5 mm diameter) were harvested from the iliac crest and morselized with an electric bone mill. After mixing with amorphous bone marrow aspirated from the depth of the biopsy areas, the osteogenic material was loaded into the central passage of the predrilled blood-soaked cylinders. Each sheep received two cylinders. The first cylinder (group 1) was implanted directly into the latissimus dorsi muscle after cell loading, using the whole organism as a natural bioreactor ( Fig. 1 ). To determine the effect of improved perfusion, the second cylinder (group 2) was additionally supplied with a central vascular bundle after surgical preparation of perforating vessels from the thoraco-dorsal trunk ( Fig. 2 ). Three months after surgery, all animals were killed after deep sedation (midazolam 1 mg/kg i.m., propofol 5 mg/kg i.v., pentobarbital 80 mg/kg i.v.) followed by conventional CT examination of the cadavers. After explantation from the latissimus dorsi muscle, the cylinders were divided into two halves and fixed in 3.5% neutral buffered formalin. One half of each cylinder was embedded in methylmethacrylate (MMA) and sectioned perpendicular to the axis of the cylinder using a modified inner-hole diamond saw. Undecalcified slices of 30 μm thickness were surface stained with alizarine-methylene blue for standard light microscopy and histomorphometric analysis. The other half of each cylinder was embedded in paraffin for immunhistochemical determination of the vascular density. Immunologic reactions in decalcified slices of 1 μm thickness were performed with the antibody Factor VIII rabbit polyclonal ® (Biocare Medical Inc., Concord, CA, USA, Cat-No. CP 039 C), the ZytoChemPlus (HRP) Polymer Bulk Kit ® (Zytomed Systems, Berlin, Germany, Cat-No. POLHRP-100) and the DAB High Contrast Kit ® (Zytomed Systems, Berlin, Germany, Cat-No. DAB5000plus). Digital images of each slide were obtained using a Zeiss AxioImager MI Microscope fitted with an AxioCam MRc digital camera and AxioVision 4.5 software (Carl Zeiss, Oberkochen, Germany). The AxioVision module MosaiX was used to scan the total specimen. Electronically created images of the entire cross-section of the cylinders provided the basis for further analysis. Total bone area and residual ceramic area were quantified using the image-analysis software analySIS 3.0 ® (Olympus Soft Imaging Solutions, Muenster, Germany). For vessel counts, three regions of interest measuring 2 mm × 2 mm were defined, representing the central, intermediate and outer areas of the cylinders ( Fig. 3 ). Five slides of every cylinder were analyzed for histomorphometric evaluation and for vessel counts, which were distributed equally throughout the divided specimen. The impact of axial perfusion on bone growth, ceramic resorption and angiogenesis within the cylinders was calculated using Student’s t -test. A probability level of adjusted P was considered statistically significant for P < 0.05. The statistical software SPSS 16.0 ® (SPSS Incorporation, Chicago, IL, USA) was used for analysis of all data.



Results
All animals survived surgery with no problems. There were no complications regarding postoperative recovery. All animals started ingestion and rumination immediately after surgery. Swelling of the thoracic wall completely resolved within a week. The site of implantation was examined daily and showed no signs of infection or rejection until death. CT examination of the cadavers showed superficial ceramic resorption of the cylinders with trabecular bone growth inside. The range of measured Hounsfield units (HU) inside the cylinders was similar to the range of values of the neighboring ribs (231–1336 versus 168–1356). Compared with the histomorphometric results, the resolution of conventional CT was not sufficient to distinguish between the trabecular and porous microstructure of bone and ceramic and therefore to determine the exact amount of bone inside the scaffold ( Fig. 4 A and B ). In group 2, the implanted vascular bundle was detectable as a radiolucent lacuna in the center of the cylinder on axial slices. Dislocation of the cylinders from the original site of implantation in the thoracic wall was not observed. In two animals, the cylinders were completely smashed. These animals were excluded from the study, leaving 10 animals with 10 cylinders for each group for further analysis. When the constructs were explanted and divided into two halves, solid and bleeding surfaces of the cut ends were found, giving macroscopic evidence for vital bone formation inside the cylinders ( Fig. 4 C). In group 2, the implanted vascular bundle was easily detectable by expression of blood from the surrounding soft tissue ( Fig. 4 D). Microscopically, vital bone formation was observed inside the cylinders, which mainly filled the center of the cylinders. Osteogenic activity was evident due to the presence of osteoblasts and osteoid seams aligned along the mineralized surfaces. The trabecular bone structure was penetrated by different amounts of fibrous tissue. Invasion of the ceramic with conversion to bone was observed only when there was a direct interface between bone and ceramic without an intervening layer of fibrous tissue. The remaining pore spaces were completely filled with highly vascularized fibrous tissue ( Fig. 4 E and F). Histomorphometric analysis revealed, that ceramic resorption ( P = 0.028) and bone formation ( P < 0.001) were significantly higher in group 2 and therefore associated with axial perfusion ( Fig. 5 A and B ). Immunhistochemical determination of the vascular density showed a significantly higher number of vessels in group 2 as a result of improved angiogenesis due to implantation of a central vascular bundle ( P < 0.001) ( Fig. 5 C). This statistical difference was still significant, when central ( P = 0.018), intermediate ( P = 0.025) and outer ( P = 0.042) regions of interest in the cylinders were examined separately.


Clinical application
Clinical application in a man was performed in consultation with the hospital ethics committee. The patient was a 57-year-old man who had suffered from chronic osteomyelitis for many years and finally underwent continuity resection with loss of his left hemimandible. The continuity defect was simultaneously bridged with a reconstruction plate. Adipositas, hypertension, diabetes mellitus (IDDM IIb) and heavy nicotin abuse were comorbidities. During follow-up, the reconstruction plate penetrated the covering soft tissue exposing the oral cavity. There were no signs of chronic osteomyelitis 1 year after surgery.
The infected reconstruction plate was removed and replaced by a new reconstruction plate through a transoral approach. Wound closure was performed with an ipsilateral nasolabial flap, creating a highly vascularized soft tissue bed. Simultaneously, three bone biopsies (5 mm diameter) were harvested from the iliac crest, morselized with an electric bone mill and mixed with amorphous bone marrow aspirated from the depth of the biopsy areas. After dissection of the latissimus dorsi muscle on the patient’s left side and preparation of perforating vessels from the thoraco-dorsal trunk, four blood-soaked cylinders were supplied with a central vascular bundle and loaded with the harvested osteogenic material. There were no complaints of pain or discomfort at the site of implantation until transplantation.
Four vascularized bioartificial bone grafts were prefabricated in vivo over 6 months. An angio-CT was produced prior to transplantation, which demonstrated perfusion of the bioartificial bone grafts through the central vascular bundle ( Fig. 6 A ). Axial vascularization was clinically confirmed during surgery when the vascularized bioartificial bone grafts were explanted, making electrocoagulative hemostasis necessary ( Fig. 6 B). The cylinders were shaped using piezo-surgery according to a virtual template ( Fig. 6 C), which was preoperatively created using Voxim-software ® (IVS solutions, Chemnitz, Germany). After exposure of the reconstruction plate through a submandibular approach, a titanium mesh (Synthes, West Chester, USA) was adapted to a commercialized mandible in order to create a bowl ( Fig. 6 D), which was filled with the individually shaped bioartificial bone grafts and fixed to the mandible with two screws on each side for stabilization. The base of the bowl and the interface between the shaped bioartificial bone grafts were covered with osteogenic material gained from the iliac crest ( Fig. 6 E and F).
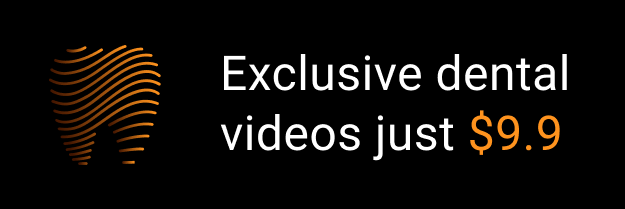