Highlights
- •
Polymerization stress increased when 3% of particles were replaced by short fibers.
- •
Polymerization stress was not affected by replacing 6% of particles with fibers.
- •
Fiber orientation imposed by specimen height did not affect polymerization stress.
- •
Flexural modulus was reduced by the replacement of particles with 3% and 6% fibers.
- •
Volumetric shrinkage was reduced only in the composite with 6% of fibers.
Abstract
Objective
To test the null hypotheses that (1) the replacement of particles by short fibers does not affect polymerization stress (PS), flexural modulus (FM) or volumetric shrinkage (VS) of experimental composites and (2) PS is not affected by specimen thickness.
Methods
Three experimental composites were prepared, each containing similar mass fractions of BisGMA and TEGDMA and 60 vol% of fillers, being 0%, 3% or 6% constituted by 1.6-mm long glass fibers and the remaining by 1 μm glass particles. PS ( n = 5) was tested in a high compliance system, using two specimen heights (1.5 mm and 4.0 mm). VS and maximum shrinkage rate were obtained in a mercury dilatometer ( n = 3). FM was tested in three-point bending ( n = 10). As an additional control, a commercial composite (Filtek Z250, 3 M ESPE) was tested. Data were recorded 10 min after the onset of photoactivation and analyzed by ANOVA/Tukey test (FM only) and Kruskal–Wallis (alpha: 5%).
Results
At both specimen heights, the composite with 3% of fibers presented significantly higher PS than the controls (which showed similar PS values). Replacing 6% of particles by fibers did not increase PS significantly. FM was reduced in the presence of fibers, and 6% of fibers led to a decrease in VS. Shrinkage rate was not affected by the fibers.
Significance
Replacing 3 vol% of particles by fibers resulted in significantly higher PS, which was associated to a decrease in FM compared to the control. PS was not affected by specimen height for any of the tested materials.
1
Introduction
Investigations on the use of fibers as reinforcing agents in dental polymeric materials started in the 1960’s, soon after fiber-reinforced polymers became available in large industrial scale . Since then, several studies have tested the effect of adding continuous glass or carbon fibers on the mechanical properties of acrylic resins and several clinical applications were devised for continuous fiber-reinforced composites, such as periodontal and orthodontic splints and fixed prosthodontics substructures .
While maximum polymer reinforcement is obtained with oriented continuous fibers, these materials are not easily molded into complex shapes . For this reason, short fibers are very often used to reinforce polymers. Unfortunately, short fibers do not provide the same level of strength obtained with long continuous fibers and, if they are randomly oriented, a four-fold lower strength is expected . Still, randomly oriented short fibers are effective polymer-reinforcing agents depending on their volume fraction, aspect ratio ( i.e. , length/diameter) and the interfacial strength between fiber and resin matrix .
In the 1990s, composites containing relatively low aspect ratio glass fibers (AR < 10) as part of the filler systems became commercially available. Though mechanically these materials did not stand out amidst particle-only materials , in one of them fibers were responsible for its “packable” viscosity . More recently, a bulk-fill composite containing filler particles and high aspect ratio E-glass fibers (17 μm in diameter and 1–2 mm in length, AR: 59–118) was developed. Its mechanical strength is comparable with those presented by particulate composites , while fracture toughness seems to be improved by the presence of fibers .
A clinically relevant, but least explored aspect of fiber reinforced composites is their polymerization shrinkage and associated polymerization stress. High-aspect ratio fiber-reinforced composites are not suitable for restoring the entire cavity due to their poor polishing, and a “cap” of particle-filled composite must be applied as the last increment. Therefore, some of the clinical problems usually associated with polymerization stress, such as marginal gaps and the development of white lines due to enamel cracking at the margins, may be less of a concern. Still, investigating their polymerization stress behavior is important in order to access, for example, a possible risk of internal gap formation and the post-operative sensitivity.
It was demonstrated that shrinkage is greatly reduced in the direction of continuous long fibers . However, the available literature shows that random short fibers may have different effects on post-gel shrinkage depending on the composite fiber content, testing method and the choice of controls . The same seems valid for polymerization stress. While one study found lower stress for an experimental fiber-reinforced composite in comparison to a commercial particle-filled control , a recent study evaluating bulk-fill composites reported significantly higher polymerization stress values and interfacial debonding for a fiber-reinforced composite .
Based on the above, the objective of the present investigation was to evaluate, in a series of experimental composites, the effect of replacing filler particles by high aspect ratio glass fibers on the polymerization stress and its determinants (i.e., volumetric shrinkage and flexural modulus). The null hypotheses were: (1) the replacement of glass particles by short fibers does not affect polymerization stress, flexural modulus or volumetric shrinkage of experimental composites and (2) composite polymerization stress is not affected by the thickness (or height) of the specimen.
2
Materials and methods
2.1
Composite formulation
Three experimental composites were prepared with a resin matrix containing equal parts by weight of bisphenol-A glycidyl dimethacrylate (BisGMA, all chemicals from Sigma–Aldrich, St. Louis, MO, USA) and triethylene glycol dimethacrylate (TEGDMA). Photoinitiators (camphorquinone and ethyl-4 dimethylamino benzoate, EDMAB) were added at 0.5% (by weight) each. S-2 glass fibers, 7–13 μm in diameter (Advanced Glassfiber Yarns, Aikin, SC, USA) were cut into 1.6-mm long segments (standard deviation: 0.5 mm) and silanized using 3-methacryloxypropyl-trimethoxysilane (3-MPTS). Fiber length distribution is shown in the histogram in Fig. 1 . Silane content on the fibers was 6%, as determined by thermogravimetric analysis. In all three experimental composites, filler fraction was 60 vol%, divided between fibers (0%, 3% or 6%) and silanized barium glass particles (D 50 : 1.0 μm). The components were mechanically mixed at 2500 rpm under vacuum for 30 s (Speedmixer DAC 150.1 FVZ-K, FlackTek Inc., Landrum, SC, USA) and kept under refrigeration until 2 h before use. A commercial composite containing 60 vol% of fillers (0.19–3.3 μm, Filtek Z250, shade A3, 3 M ESPE, St Paul, MN, USA) was used as an additional control.
2.2
Polymerization stress test
As bonding substrate for the composite, 5-mm diameter acrylic rods were sectioned in 28-mm long segments and had one of their flat surfaces sandblasted. Prior to the test, methyl methacrylate was applied to the sandblasted surfaces to improve wetting by a thin layer of unfilled resin (Scotchbond Multipurpose Plus, 3 M ESPE). The unfilled resin was light-cured for 10 s (Radii-cal, SDI, Victoria, Australia). The rods were attached to the opposite fixtures of a universal testing machine (Instron 5565, Canton, MA, USA) and positioned at pre-set distances of 1.5 mm or 4.0 mm. The composite was inserted between both rods and shaped into a cylinder following their external circumference. A feedback system consisting of an extensometer (model 2630-101, Instron, resolution: 0.1 μm) was used to keep specimen height constant throughout the test. The extensometer sensors were clamped to both rods and any change in specimen height due to composite shrinkage immediately commanded the crosshead to restore the initial distance between rod surfaces. Composite was light cured for 20 s using two curing units (Radii-cal, SDI), positioned on opposite sides of the specimen. According to manufacturer’s information, each unit delivered an irradiance of 1,200 mW/cm 2 . Shrinkage force was monitored for 10 min and maximum nominal stress was calculated dividing the highest force value recorded by the cross-section area of the rod. Five specimens were tested per group.
2.3
Volumetric shrinkage
Composite volumetric shrinkage was measured in a mercury dilatometer (ADA Health Foundation, Gaithersburg, MD, USA). Approximately 0.15 g of composite was placed on a sandblasted, silanated glass slide and shaped as a hemisphere. The glass slide was clamped to a glass column, which was then filled with mercury. An LVDT probe (linear variable differential transducer) was positioned touching the mercury surface. Photoactivation was conducted with the tip of the light guide placed underneath the glass slide. LVDT readings were converted to volumetric shrinkage values using input values of composite mass and density. The decrease in mercury column height due to composite shrinkage was monitored for 60 min, at the rate of 0.1 s −1 . Statistical analysis used shrinkage values recorded 10 min after the beginning of photoactivation. Maximum shrinkage rate (in % s −1 ) was calculated as the first derivative of the shrinkage versus time curve, as an indication of polymerization reaction rate. Three specimens were tested in each group.
2.4
Flexural modulus
Flexural modulus was calculated based on the load versus displacement curve obtained in three-point bending testing. Bar-shaped specimens (10 mm × 2 mm × 1 mm, n = 10) were built using a stainless steel split mold. A single 20-s exposure was used to cure the specimen (Radii-cal, SDI). Ten minutes after the beginning of photoactivation, the specimen was removed from the mold and placed on a testing jig positioned under the crosshead of a universal testing machine (Instron 5565), with an 8-mm span between the supporting rollers and the irradiated surface on the compression side. Crosshead speed was set at 0.5 mm/min. Displacement of the central portion of the specimen was recorded by a deflectometer (model W-E401-E, Instron). Flexural modulus was calculated using data obtained from the initial portion of the load versus displacement curve, according to the following equation:
E = C × L 3 4 × b × h 3 × d × 10 − 3
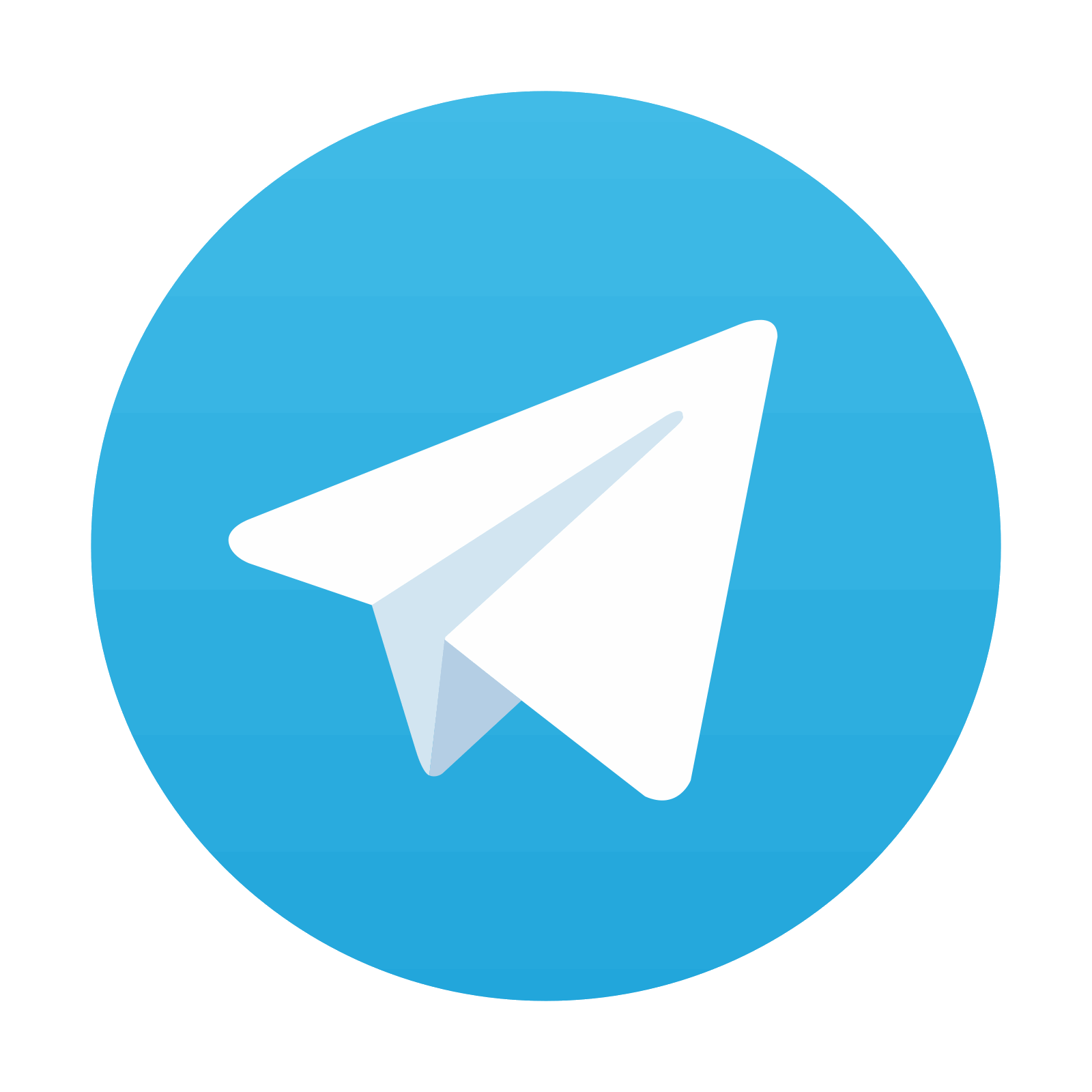
Stay updated, free dental videos. Join our Telegram channel

VIDEdental - Online dental courses
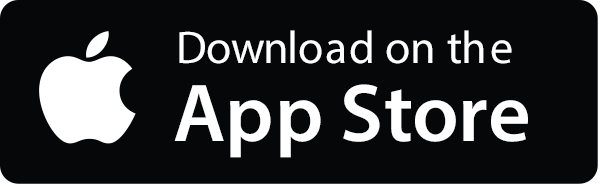
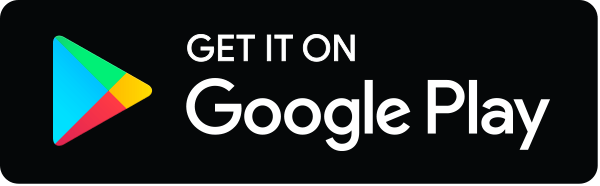