27
Photofunctionalization in the Treatment of Peri‐implantitis
Robert Miller
Department of Oral Implantology ACDRC, Palm Beach State College, FL, USA
The acceptance of oral implantology as the standard of care in oral reconstruction has led to an exponential increase in the use of dental implants by clinicians of all specialties. Concurrent with this increased use of implantable devices has been the increased incidence of peri‐implantitis [1]. While the causes of peri‐implantitis are varied, the one common thread has been alterations in the surface characteristics of implanted devices, and many different treatment modalities have been suggested for treatment of these implant site defects [2]. However, treatment of peri‐implantitis can be refractory, with a significant number of treatment attempts failing to achieve the desired results [3, 4].
The conditions leading to the development of peri‐implantitis are multifactorial, and there is one aspect of peri‐implant disease that is not well understood. That parameter is the condition of the implant surface. Metallic oxide surfaces are quite complex, and they exist in a range of electron states that affect the quality of fluid and tissue adhesion [5]. When titanium or zirconium implants are manufactured, the oxide layers that immediately form are pristine and, consequently, extremely hydrophilic and electrostatically active [6]. Within days of manufacture, however, inorganic ions from the atmosphere start to populate the active receptor sites on these oxide layers [7]. Implant surfaces undergo a fundamental change, becoming less electrostatically active and convert to a hydrophobic form (Figure 27.1). The term “biologic aging of titanium” has been coined to describe the time‐related degradation of physiochemical properties of implant surfaces, with a direct effect on the ultimate quality and quantity of bone at the implant interface.
This will diminish the degree of protein and fibrin attachment and may affect the quality of osseointegration [8]. (Figures 27.2 and 27.3) A lower quality of fibrin adhesion may delay cell migration and result in a lower percentage of bone‐to‐implant contact. Early implant failures may be related to this phenomenon.
In addition, implants that suffer from peri‐implant disease are not only contaminated with inorganic ions, but there is now a layer of organic contamination that potentially complicates treatment outcomes. Strategies to treat peri‐implantitis have previously focused on removal of organic bioburden and decontamination. We now recognize that treatment of the oxide surface at the molecular and atomic levels is now becoming an integral part of the treatment protocol to ensure reintegration of the implant body.
A critical factor in implant integration is biocompatibility, and successful integration and survival of dental implants in function are directly related to the percentage of bone‐to‐implant contact. It is reported that percentage of vital bone in successfully integrated implants ranges from 45 to 65% [9]. Early failure of dental implants is most often related to insufficient load‐bearing bone, resulting in breakdown and resorption of the remaining bone complex. Therefore, biocompatibility becomes the most important factor in forming the highest percentage of vital bone in direct apposition to the implant surface.
There are several physiochemical properties that contribute to biocompatibility, which includes surface topography, hydrophilicity, and chemical composition [10]. Morra et al. commenced a study of 34 different implant systems and analyzed hydrocarbon deposition on titanium oxide surfaces. They found a range of 17.9–76.5% carbon deposition [11]. This hydrocarbon contamination existed on all implants tested, regardless of surface characteristics.
Early failures of dental implants may be directly related to a low percentage of bone‐to‐implant contact. These percentages under normal conditions may range from 50 to 65% [12].

Figure 27.1 Hydrophobic implant surface repelling fibrin attachment.
(Source: Reprinted courtesy Professor Dr. Nelson R. Pinto, Department of Periodontics and Implant Dentistry, Faculty of Dentistry, University of the Andes, Santiago, Chile).
Surface Characteristics of Metallic Oxide Surfaces
Studies have shown that the electron state of metallic oxide surfaces can affect protein absorption, and osteoblast migration, differentiation, and proliferation.
Surface analysis of implant surfaces can be accomplished by using SEM (Scanning Electron Microscope), AES (Auger Electron Spectroscopy), and XPS (X‐ray Photoelectron Spectroscopy). These instruments can perform elemental analysis of the oxide surfaces to measure both morphology and chemistry. Within the titanium oxide or zirconium oxide surfaces, we can detect hydrocarbon contamination of the oxide receptors and ultimately test the affinity of these surfaces for biologic fluids and cell attachment [13]. The primary contaminant is carbon, with smaller percentages of nitrogen, oxygen, aluminum, and fluoride.


Figures 27.2–27.3 Poor quality fibrin adhesion on aged titanium.
(Source: Reprinted courtesy Professor Dr. Nelson R. Pinto, Department of Periodontics and Implant Dentistry, Faculty of Dentistry, University of the Andes, Santiago, Chile).
In a study by Roy et al. they measured contaminant deposition corresponding to the presence of carbon‐containing molecules on implant surfaces. The peak attachment occurred at 285 eV for all species, and then the population of these molecules was measured after UVC irradiation. They found that the percentage of hydrocarbon contamination decreased by a factor of 4X. Another peak at 534 eV was found for contamination by H20. Photofunctionalization was found to decrease the H20 concentration and covert the receptor site to TiOH, resulting in an increase in hydrophilicity by changing the electron charge of the surface [14]. (Figure 27.4) Changing the binding energy (eV) of titanium receptors will result in the uncoupling of hydrocarbon contaminants and conversion to electropositive species that attract biologic fluids. (Figure 27.5)
It is hypothesized that, in the biologic model, additional contamination will occur through the attachment of lipopolysacharrides, bacterial endotoxins, calculus, and dental plaque. Debridement of the implant surface must precede photofunctionalization. This can be accomplished by using ultrasonic instrumentation, chemotherapeutic agents, citric acid, prophyjet, or ablative lasers [15]. Following removal of the bioburden on the implant surface, UVC energy can then reach the oxide layer [16]. The final removal of organic and hydrocarbon residues results in a change in electrostatic condition, allowing adsorption of organic components in biologic fluid, primarily carboxyl, and amine groups. This early phenomenon now facilitates adhesion and migration of regenerative cells, resulting in integration of the implanted device [17–19] (Figure 27.6).

Figure 27.4 The core lines recorded for as received and after photofunctionalization samples. The lines in red show the decontamination effect of the UVC irradiation decreasing the hydrocarbons peak (a) and increasing the oxygen peak (b). The red lines demonstrate the decontamination effect of UVC irradiation by decreasing hydrocarbon peaks and increasing oxygen binding.
(Source: Reprinted courtesy Roy et al. [14]).

Figure 27.5 Comparing the intensity of the peak at 285 eV corresponding to carbon contamination and oxygen binding before (a, c) and after (b, d) UVC irradiation.
(Source: Reprinted courtesy Roy et al. [14]).
Osteoblast Attachment and Migration
Adhesion and motility of osteoblasts can be affected by surface properties of implant surfaces [20]. Reduction of the ability of osteoblasts to adhere to the implant surface and reduction of cell spreading across that surface has a direct effect on the production of signaling proteins in the osteoblast. These Rho GTPases help to regulate actin fibers in the osteoblast and are responsible for cell mobility. Any reduction of these signaling proteins will interfere with cell attachment and spreading. As hydrocarbon contamination increases, there is a linear reduction in cell migration and spreading. Photofunctionalization helps to indirectly stimulate GTPase gene expression, upregulating osteoblast metabolism [21] (Figure 27.7).

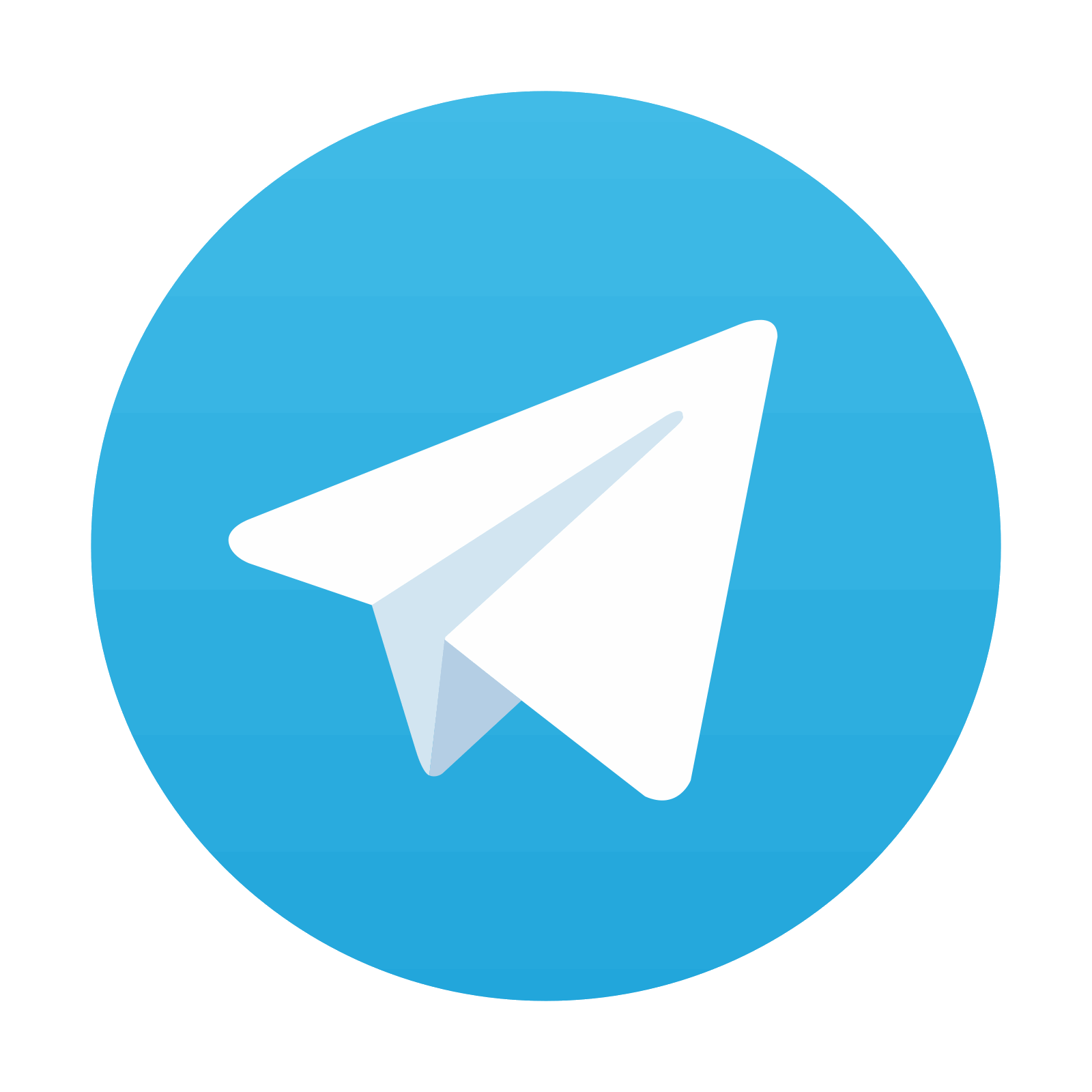
Stay updated, free dental videos. Join our Telegram channel

VIDEdental - Online dental courses
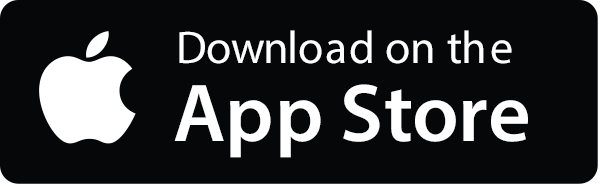
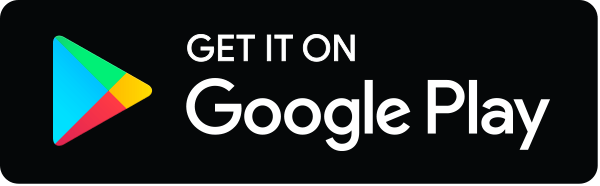