Abstract
Objective
This review surveys new developments in bone tissue engineering, specifically focusing on the promising role of nanotechnology and describes future avenues of research.
Methods
The review first reinforces the need to fabricate scaffolds with multi-dimensional hierarchies for improved mechanical integrity. Next, new advances to promote bioactivity by manipulating the nanolevel internal surfaces of scaffolds are examined followed by an evaluation of techniques using scaffolds as a vehicle for local drug delivery to promote bone regeneration/integration and methods of seeding cells into the scaffold.
Results
Through a review of the state of the field, critical questions are posed to guide future research toward producing materials and therapies to bring state-of-the-art technology to clinical settings.
Significance
The development of scaffolds for bone regeneration requires a material able to promote rapid bone formation while possessing sufficient strength to prevent fracture under physiological loads. Success in simultaneously achieving mechanical integrity and sufficient bioactivity with a single material has been limited. However, the use of new tools to manipulate and characterize matter down to the nano-scale may enable a new generation of bone scaffolds that will surpass the performance of autologous bone implants.
1
Introduction
Bone is one of the most commonly transplanted tissues with 2.2 million bone grafts performed annually worldwide . Indeed, surgeons face a diverse spectrum of clinical challenges in bone reconstruction reflecting the variety of anatomic sites, defect sizes, mechanical stresses, and available soft tissue cover (see Table 1 ). Autologous bone grafting remains the gold standard for reconstruction of skeletal defects ; however, this technique does have some drawbacks including limited supply, bone graft loss/resorption, short-term instability in large defects, complications associated with a second surgical site, and autograft failure rates exceeding 50% in difficult healing environments . Bone allografts (i.e. bone transplanted from a donor) present another option, accounting for about one-third of bone grafts performed in the United States . However, their clinical success does not approach that of autologous bone, with higher failure rates (30–60% over 10 years in vivo with significant decreases in strength) and prevalence of late rejection .
Functional requirement Potential clinical application |
Mechanical requirements | Bone formation | Candidates | Benefits of appropriate scaffolds | ||
---|---|---|---|---|---|---|
Strength | Young’s modulus | Material | Form | |||
Low load-bearing • Sinus augmentation • Extraction sites |
Minimal | 0.05–1 GPa | 4 months | • Polymers • Polymer/ceramic hybrid composites |
• Injectable • Flexible |
• Eliminates risks of bone grafting • Eliminates xenogeneic materials and potential inflammatory response • Ease of handling |
Medium load-bearing • Alveolar cleft repair • Alveolar ridge augmentation • Calvarian repair |
>50 MPa | 1 GPa | 4–5 months | • Polymers • Polymer/ceramic hybrid composites |
• Some density to support soft tissue or limit soft tissue collapse • Porous matrix |
• Eliminates risks of bone grafting • Ease of handling • Shortens surgical time |
High load-bearing • Segmental mandibular defect repair • Segmental tibial defect repair • Cervical disk |
>150 MPa | 10–30 GPa | >6 months | • Inorganic • Hybrid composites with high mineral content |
• Porous matrix • Dense: able to create porosity in vivo |
• Eliminates risks of bone grafting or flap surgery • Shortens surgical time • Rapid return of function |
As a result of these limitations, the use of synthetic implants to replace damaged bone is growing exponentially. However, current synthetic biomaterials were developed originally for other engineering applications and often do not integrate well with host tissue resulting in possible infection, foreign-body reactions, and extrusion/loss of the implanted material. While current biomaterials result in a time-limited and unpredictable outcome , an alternative that has attracted widespread attention in recent years is the engineering of new bone to replace the damaged or diseased tissue. A critical component of this tissue engineering approach is the development of porous 3D structures – scaffolds – that will provide cell support and guide bone formation. Numerous porous materials have been investigated, but despite substantial progress in the field, the development of synthetic structures able to fully harness the bone’s capability to regenerate and remodel itself still presents challenges.
Autologous bone grafts owe their success to the presence of endogenous bioactive molecules and cells able to respond to the signals in the graft and the surrounding microenvironment. A successful bone engineering therapy must recapitulate, and ideally accelerate, the process of bone regeneration, which will only be possible if we understand the complex process of bone healing and identify the critical steps. Following the example of autologous grafts, most bone engineering approaches are based on the combination of four factors: a matrix (i.e. the scaffold), cells to “build” the new tissue, cell signaling (BMPs and growth factors) to guide cell differentiation and tissue formation, as well as an adequate blood supply (i.e. vascularization) (see Fig. 1 ). Thus, there are multiple physical and biological requirements that an ideal bone scaffold should address: (i) supply a porous matrix with interconnected porosity and tailored surface chemistry for cell growth, proliferation, and transport of nutrients and metabolic waste; (ii) resorb/remodel in a predictable way with controlled osteogenic activity and produce only metabolically acceptable substances; (iii) deliver a controlled cascade of signaling (both in time and space) to guide cell differentiation and promote tissue regeneration; (iv) match the mechanical properties of the host tissues with a strong, stable material-tissue interface persisting through the implant resorption process; (v) eliminate the risk of rejection or foreign-body reaction; and (vi) achieve good adaptation and coverage by the surrounding soft tissue. By meeting these requirements, the implant can substitute, at least temporarily, for natural tissue, providing sufficient strength and stiffness to prevent fracture under physiological loads and provide a framework for the body to create new bone tissue.
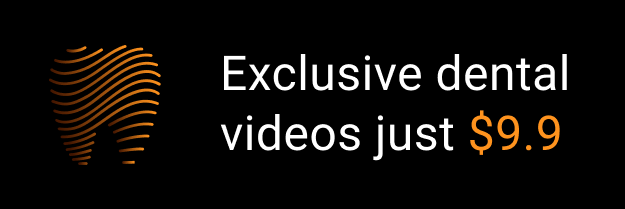