10.1055/b-0034-84405
The Disease: 2 Etiology and Pathogenesis of Caries
As described in Chapter 1, teeth are continuously bathed in saliva, one of the main functions of which is to minimize mineral dissolution and precipitation within the mouth1,2: both can be harmful to the teeth and other oral tissues. Saliva can ameliorate the effects of challenges that tend to dissolve tooth tissue, such as consumption of acidic foods, because it has an approximately neutral pH, is reasonably well buffered, and contains mineral ions. In dental caries, this homoeostasis is overcome by acid-generating metabolic processes in localized accumulations of bacteria. This in turn causes loss of mineral from the hard tissues, which destroys their integrity and eventually impairs their function.
Most of the surface of a tooth is kept free of bacteria by friction from the tongue, cheeks, and foodstuffs. However, bacteria colonize areas of the surface protected from these frictional forces (plaque stagnation areas) and form a film of closely packed bacteria known as dental plaque 3,4 within which is created a unique microenvironment, partly isolated from the saliva and immediately adjacent to the tooth surface. The human diet includes a variety of easily-fermentable carbohydrates: monosaccharides such as glucose and fructose; disaccharides such as sucrose and maltose; and oligosaccharides such as those found in honey. In this chapter, these will be collectively referred to as ‘sugar’ and specific carbohydrates will be named. On each occasion when sugars are ingested, they are metabolized by plaque bacteria and this results in the accumulation of organic acid end products and hence causes a temporary reduction in plaque pH. Such an episode can pose a “cariogenic challenge” since, if the plaque pH falls low enough, mineral within the underlying dental hard tissue can dissolve. The progressive loss of mineral through dissolution by plaque acid (demineralization) during repeated cariogenic challenges is the primary process in dental caries.
This basic etiology is summarized by the well-known Venn diagram of Keyes5 ( Fig. 2.1 ), which illustrates the interaction of the three factors “tooth,” “bacteria,” and “diet.” While the combination of two factors will produce a contribution (e.g., bacteria + tooth → plaque; bacteria + diet → acid), the interaction of all three is required for caries initiation. Lesions are initiated only at sites where plaque accumulates. In economically developed populations, primary caries lesions are initiated in children on the enamel surface: most commonly in occlusal pits and fissures, less often on approximal surfaces, and rarely on smooth surfaces. In young adulthood, approximal caries increases. In older people, root surfaces exposed by gingival recession are sites for new primary lesions, and the margins of restorations are sites for secondary or recurrent caries lesions.6
Caries tends to progress relatively slowly (over months or years) and in the early stages demineralization produces subsurface lesions which can in principle be arrested or reversed. Between cariogenic challenges, plaque pH returns toward “resting” levels which are approximately neutral, and this allows the possibility that mineral ions in plaque can contribute to re-deposition of mineral within the caries lesion: a process known as remineralization. Thus, the caries process is not one-directional but involves a dynamic process of mineral loss and regain7 ( Fig. 2.2 ). If the balance between these processes favors demineralization, caries lesions progress and ultimately the damage to the tissue, due to mechanical breakdown (enamel) or to bacterial action (dentin), becomes irreversible. Restoration or extraction then becomes the only treatment option.

Despite this apparently simple etiology, caries is regarded as a multifactorial disease, for two main reasons. First, despite much research, it has not been proven whether it is caused by one specific pathogen or by several bacteria. Second, the risk of caries occurrence, and the rate at which the disease progresses, are influenced by a large number of factors.6,8 These form a hierarchy at individual, behavioral, and social levels:
- Individual factors: the oral bacterial flora; the solubility of tooth mineral; hard tissue structure; salivary flow rate and composition
- Behavioral factors: frequency with which foods containing fermentable carbohydrate are consumed; frequency and effectiveness of oral hygiene; pattern of dental check-ups
- Social factors, such as level of education and socioeconomic status, influence aspects of individual behavior which impinge on caries. Caries incidence in children is strongly influenced by the level of care provided by those looking after them, especially with respect to diet, attention to oral hygiene, and attendance at the dentist. Care provision is in turn influenced by the care-giver′s background.

Caries is profoundly influenced by exposure to fluoride, which reduces demineralization and enhances remineralization.7,9 As fluoride is most often delivered in the form of toothpaste, social and behavioral influences on oral hygiene habits take on a special significance (see Chapter 13).
The aims of this chapter are to outline the relevant biological and chemical factors, and to describe their interactions in caries initiation and progression. In particular this chapter will cover:
- Microbiology: changes in plaque flora associated with caries
- Chemistry of dental minerals: concepts of solubility, dissolution and crystal growth; properties of tooth minerals; the effects of fluoride
- The cariogenic challenge: properties of dental plaque; acid production from sugar and its effect on pH; factors influencing severity of the cariogenic challenge
- Chemistry of caries: physicochemical processes controlling lesion formation and arrest
Caries is not the only demineralization-related oral disease. Dental erosion, caused by direct action of acids on tooth surfaces without bacterial action, results in increased wear.10 A brief discussion of dental erosion concludes the chapter.
Microbiology of Caries
In his chemo-parasitic theory (1890), Miller postulated that caries was caused by acids produced in the mouth by bacteria metabolizing dietary carbohydrate in food particles retained between the teeth.68 Until the 1960s lactobacilli were favored as the likely pathogens because they are highly acidogenic (capable of rapidly converting sugar to acid) and aciduric (capable of withstanding low-pH conditions). Then, from a series of classic experiments with rodents, it was concluded that caries was an infectious, transmissible disease11 and attention shifted to streptococci, especially Streptococcus mutans, first isolated in 1928. Besides being acidogenic and aciduric, S. mutans synthesizes an insoluble, sticky extracellular polysaccharide from sucrose which promotes adhesion of the organism. Since the 1960s, an enormous body of research on the microbiology of caries has accumulated and many observational, longitudinal, and intervention studies have provided strong evidence for an association of S. mutans with caries.12 Indeed, many workers have concluded that S. mutans is the sole pathogen involved in caries. Usually, this is extended to include other members of the taxonomic group to which S. mutans belongs—the mutans streptococci (see Chapter 1)—particularly S. sobrinus, which is also isolated from cariogenic plaque of humans, although less frequently and in smaller numbers than S. mutans. The hypothesis that caries is caused by infection with S. mutans or the mutans streptococci, is known as the specific plaque hypothesis.13
However, S. mutans usually makes up only a very small proportion of the plaque flora, is not always detectable in plaque associated with caries, and can occur in plaque without caries developing.14 Further, while S. mutans is particularly acidogenic and aciduric, these properties are also exhibited to some extent by a variety of plaque bacteria. These include not only S. sobrinus but several “low-pH” members of the Streptococcaceae, such as strains of S. oralis. Other acidogenic/aciduric plaque bacteria include strains of Actinomyces, such as A. israelii and A. gerencseriae, bifidobacteria, and lactobacilli. Recognition of this fact underlies the nonspecific plaque hypothesis, which suggests that acidogenic, acid-tolerant bacteria besides S. mutans contribute to the caries process and, in the absence of S. mutans, could be the sole agents of caries initiation.14,15
A third hypothesis, the ecological plaque hypothesis, emphasizes the importance of the oral environment in determining the composition and properties of the plaque microflora.16 According to this hypothesis ( Fig. 2.3 ), in the mouths of persons consuming a low-sugar diet the plaque bacteria would derive their energy predominantly from slow breakdown of complex salivary and dietary molecules, so would experience only small and infrequent drops in pH. An increased frequency of sugar intake disrupts the homoeostasis of such a plaque because it favors growth of acidogenic, aciduric bacteria and hence promotes low-pH conditions. Bacteria which are sensitive to low pH grow less well under these conditions and are selected against. Thus an increased availability of sugar causes an ecological shift in the plaque microflora which establishes caries-conducive conditions. Since bacteria are selected solely on the basis of their ability to produce acid and to withstand low pH, this process is nonspecific and the bacteria which increase in a high-sugar environment can include a range of species, as noted above. However, if S. mutans has colonized the mouth its growth will certainly be favored, especially under conditions of very high sugar intake, which will result in the creation of extremely acidic conditions which give this species a competitive advantage. Such a sugar-rich, low-pH environment would also favor colonization by lactobacilli and by the fungus Candida.
The ecological plaque hypothesis is supported by considerable evidence. The microflora of plaque from the approximal region is complex and dominated by Gram-positive, rod-shaped bacteria (mainly Actinomyces) and streptococci, of which the most abundant is typically S. sanguinis, with smaller proportions of other bacteria, such as Bacteroides, Neisseria, Veillonella, Fusobacterium, Rothia, and Lactobacillus. However, the composition of the flora varies considerably between different sites on the tooth surface. An increased sugar intake results in a higher proportion of acidogenic/aciduric bacteria,17 and increases in S. mutans, Lactobacillus, and others have been observed, along with a decrease in the less acid-tolerant S. sanguinis 18 ( Fig. 2.4 ). Plaque from caries-active people has a higher proportion of acidogenic bacteria than that from caries-free people: these changes affect plaque in general, not just on surfaces on which lesions form.19 Changes in the plaque flora can be difficult to identify because of extensive intra- and inter-individual variation, but in caries-active people the proportion of S. sanguinis and of Actinomyces naeslundii typically fall. During the initial stages of caries, the abundance of S. mutans, S. oralis, acidogenic actinomyces, such as A. gerencseriae, and lactobacilli increase. In advanced (cavitated) lesions, there may be moderate increases in S. mutans, but the flora is dominated by lactobacilli, Bifidobacterium, and Prevotella.20,21

Proponents of the specific plaque hypothesis recognize the powerful ecological effect of dietary sugar in determining the composition of plaque microflora, but would argue that only the increases in abundance of S. mutans are etiologically significant. However, while there is little doubt that S. mutans is a major agent of caries initiation,22 it is very likely that other acidogenic/aciduric bacteria play important roles in both initiation and progression of lesions.14,15

NOTE
Caries is probably not a “classical” infectious disease, that is, one caused by a specific bacterium not normally found in the body. Instead, it is probably due to over-growth of acid-producing, acid-resistant members of the normal oral flora, driven by excessive consumption of sugars. However, some species, especially Streptococcus mutans, do have a prominent, well-documented role in caries etiology.
Chemistry of Dental Minerals
Solubility, Dissolution, and Crystal Growth
The processes underlying the phenomena of demineralization and remineralization in caries are crystal dissolution and precipitation. In caries, the latter process is usually manifested as re-growth of partly-dissolved crystals, although precipitation of new crystals can occur. Dissolution and crystal growth are both surface-related processes. At the surface of a solid immersed in an aqueous solution (e.g., enamel crystals bathed in saliva), ions are constantly detaching from the surface and entering the solution and other ions are following the reverse path to become incorporated into the solid (A and B in Fig. 2.5 ). When the rates of these processes are equal, the solid is in equilibrium with the solution and no net dissolution or crystal growth will occur. In this situation, the solution is said to be “saturated” with respect to that particular solid. The concentration of dissolved solid in a saturated solution is a measure of the solid′s solubility. When the solution contains less than the equilibrium concentration of dissolved solid it is said to be undersaturated and when the concentration of dissolved solid in solution is greater than at equilibrium, the solution is supersaturated. When in contact with an undersaturated solution, the rate of ions leaving the solid will tend to exceed that of ions leaving the solution. This means that the solid will tend to dissolve and that crystal growth is not possible. In a supersaturated solution, crystal growth will tend to occur, as more ions leave the solution and are added to the surface of the solid, but the opposite process of dissolution cannot take place. In this description, the use of “will tend to” rather than “will” is deliberate. The reason is that in the complex environment of the mouth, both dissolution and crystal growth can be heavily influenced by another surface-related process: adsorption to the crystal surface of ions or molecules that inhibit movement of ions between the solid and the solution (C in Fig. 2.5 ). Inhibitory substances, including macromolecules such as peptides and proteins (e.g., statherin) and low-molecular-weight substances such as the pyrophosphate ion, abound in biological fluids,23 including saliva1,2 and the interstitial fluid of plaque.

In the foregoing an empirical definition of solubility is given. This is adequate for understanding the basis for demineralization and remineralization in caries, but it is important to understand that there exists a more sophisticated approach, based on fundamental principles of physical chemistry.24 This approach is more generalized and allows predictions about dissolution and crystal growth in complex systems to be made. For example, it is possible to define quantitatively the state of a given solution with respect to dissolution and crystal growth of all possible solids by calculating for each one the degree of saturation (DS). This has a value of 1 in saturated solutions, >1 in supersaturated and <1 in undersaturated solutions. Furthermore, the greater the difference between the DS and the value of 1, the greater the potential chemical driving force for the respective process. However, it is difficult to exploit this approach fully because of uncertainties in defining the solubilities of the impure minerals found in dental tissues.
NOTE
Dental minerals are impure forms of a calcium phosphate—hydroxyapatite. They become rapidly more soluble as the pH of the aqueous environment falls. Hence, teeth lose mineral in response to pH falls due to acid production in plaque and can gain mineral when the pH rises again. Fluoride reduces solubility and dissolution of tooth mineral and promotes hydroxyapatite crystal growth, so exerts powerful preventive effects on the caries process.
Minerals of Dental Tissues
Dental hard tissues are composite materials in which crystals of mineral are intimately associated with an organic matrix. The mineral is a form of hydroxyapatite, a type of calcium phosphate which in its pure form has the formula Ca5(PO4)3OH, and is the least soluble nonfluoridated calcium phosphate at neutral pH.24 Hydroxyapatite belongs to a family of minerals (apatites) which share a similar crystal structure that is remarkable for its capacity for accepting substitutions of one ion for another.25,26
The composition of hydroxyapatite in dental hard tissues is altered by incorporation in the crystal structure of several “impurity” ions, especially magnesium, sodium, and carbonate ( Fig. 2.6 ; Table 2.1 ), which originate from the tissue fluids during tooth formation. Impurity ions differ—in charge, size or both—from the Ca2+, , or OH− ions which they replace ( Fig. 2.6 ). These misfits disturb crystal structure and this in turn increases solubility. The exception to this rule is the fluoride ion, which both improves crystallinity and reduces solubility (see below). Enamel mineral contains fewer impurities than the mineral of dentin or cementum, the crystals are larger and more perfectly formed. Accordingly, enamel is only slightly more soluble than pure hydroxyapatite, while dentin is significantly more soluble,27 although a reliable solubility has yet to be established.

The solubility of a particular form of calcium phosphate, as defined by the concentration of dissolved solid in a saturated solution, is not constant but varies according to the solution composition. The dominant factor in calcium phosphate chemistry is pH: the solubility of all calcium phosphates increases as the pH falls below 7. The solubility of hydroxyapatite increases particularly rapidly with pH, by a factor of ca. 10 per pH unit fall ( Fig. 2.7 ).
Constituent | Hydroxyapatite | Enamel | Dentin |
Calcium |
39.9 |
37.6 |
40.3 |
Phosphorus | 18.5 | 18.3 | 18.6 |
Carbonate |
4.1 |
6.5 |
|
Sodium | 0.7 | 0.1 | |
Magnesium |
0.2 |
1.1 |
|
Fluoride | 0.01 | 0.07 |
Fluoride and Calcium Phosphate Chemistry
Fluoride has profound effects on solubility of hydroxyapatite and dental minerals. It is readily incorporated into the apatite structure because F− ions can replace OH− ions. Fluorapatite, in which all OH− is replaced by F−, is less soluble than hydroxyapatite at pH7 or below ( Fig. 2.7 ). In hydroxyapatite crystals, OH− ions are located within channels formed by triangular groups of Ca2+ ions, but lie between the triangles25,26 (A in Fig. 2.8 ). In fluorapatite, the slightly smaller F– ion fits within the triangles (B in Fig. 2.8 ) and this is associated with a denser, more stable crystal structure. Partial substitution of F− for OH− produces fluorhydroxyapatites, which are thought to be stabilized by hydrogen-bonding between adjacent F− and OH− ions24 (C in Fig. 2.8 ).
Important though they are, the effects of fluoride on crystal structure are probably much less significant than the reactions which take place between crystal surfaces of apatites and fluoride dissolved in the bathing liquid. If fluorhydroxyapatite, or even pure hydroxyapatite—which of course contains no fluoride ions—is placed in an acidic solution containing low concentrations of fluoride, the rate of dissolution is lower than in one that is fluoride-free28,29 ( Fig. 2.9 ). This phenomenon is due to replacement of OH− ions by F− ions at the crystal surfaces, probably not much deeper than one unit cell thickness.30 The F− ions stabilize the surrounding Ca2+ ions.30 Regions of the crystal surface in which F− has replaced OH− are in effect converted into fluorapatite, so are much less soluble than nonfluoridated regions.28,31,32 When exposed to acidic conditions, nonfluoridated regions of the crystal surfaces dissolve at the rate normal for hydroxyapatite, while the fluoridated regions will dissolve more slowly or not at all. The overall rate of dissolution is therefore slower than in the absence of fluoride, and the higher the concentration of fluoride in the ambient solution the greater the effect, because a greater proportion of the crystals surfaces is converted to fluorapatite. Thus, dissolution of tooth mineral can be partly or wholly prevented by low concentrations of fluoride in the environment of the tooth. A similar effect is thought to be responsible for the reduced solubility of fluorhydroxyapatites in fluoride-free acid.33 Here, partial dissolution of the solid produces enough fluoride ions to convert the crystal surfaces to fluorapatite.32



The lower solubility of fluorapatite also affects crystal growth. If fluoride ions are available in a solution supersaturated with respect to hydroxyapatite, crystal growth is accelerated. The solid that forms will not be hydroxyapatite but fluorapatite or a fluorhydroxyapatite, depending on how much fluoride is available, so the product of remineralization in the presence of fluoride tends to be less soluble than the mineral which had been lost.7
If teeth are exposed to high concentrations of fluoride, for example, by treatment with fluoride varnish, a form of calcium fluoride (CaF2), probably combined with phosphate, may be precipitated on the tooth surfaces.31 The Ca2+ ions required for precipitation of the CaF2-like material are derived from the tooth mineral, so more of the precipitate is formed at lower pH. Although its formation removes Ca2+ ions from the tooth surface, CaF2-like material could have a beneficial effect: since it is relatively soluble, it can act as a fluoride reservoir, maintaining raised concentrations of Ca2+ and F− ions in the tooth environment.
On the basis of this evidence, the prevailing current opinion is that strategies for caries prevention using fluoride should be aimed at maintaining low but sufficient concentrations of fluoride ions in the environment of the tooth, rather than at increasing the fluoride concentration in the tooth mineral.31 This is achieved by topical methods of fluoride administration such as toothpastes, mouth rinses, varnishes, and also by water fluoridation which, even though originally intended to reduce solubility of tooth mineral, has a significant topical effect34 (see Chapter 12).
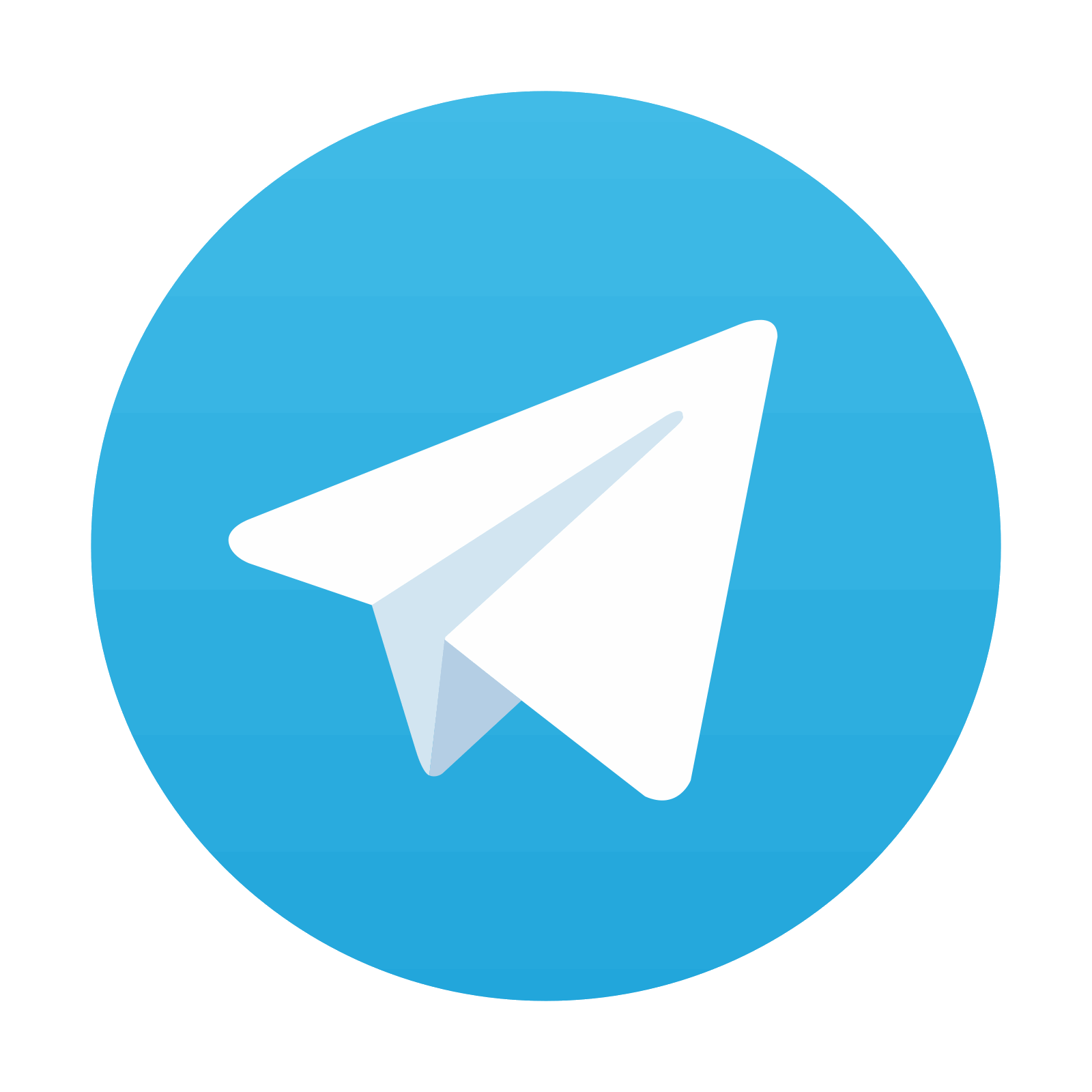
Stay updated, free dental videos. Join our Telegram channel

VIDEdental - Online dental courses
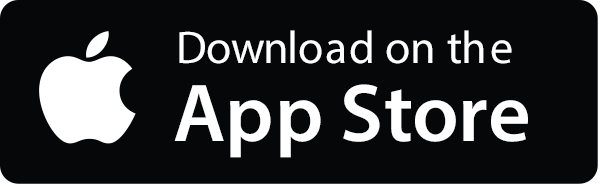
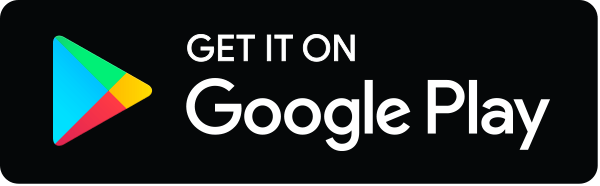
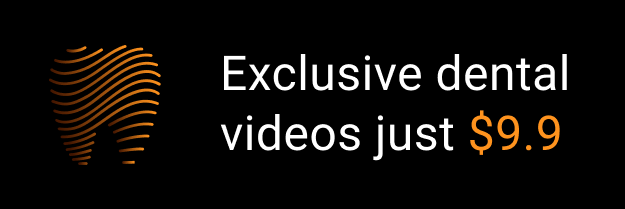