The general boundaries to tooth movement are within the adjacent compact and trabecular bones, gingiva, mucosa, and muscular envelope. Findings from finite element analysis of maxillary posterior teeth distalization against mini-implants suggest that stiff outer and interproximal compact bone resists tooth movement, regardless of bone thickness, and that teeth should be steered away from this bone during orthodontic treatment. However, individual variation in the tooth-bone interface dictates the course and outcome of treatment, offering the basis for inferences on the limits of mini-implant anchorage and the presumed influence of the regional acceleratory phenomenon through decortication and microperforation, 2 modalities advocated to effect faster tooth movement.
Highlights
- •
Variation in the tooth-bone interface dictates the course and outcome of treatment.
- •
Efficacy of mini-implant anchorage during molar distalization varies.
- •
Localized anatomic barriers in the molar region affect anchorage.
- •
Regional acceleratory phenomenon is likely involved in the healing of decortication.
- •
Acceleration of remodeling around the tooth being moved may be less important.
Orthodontic tooth movement can reach remarkable amounts: molars have been distalized 8 mm with headgear, canines moved the entire space occupied by extracted premolars or through significant impactions, and incisors retracted more than 10°. However, biologic boundaries limit tooth displacement: the housing bone, its immediate gingival and mucosal envelope, and the functioning musculature. We aimed to expose the prevailing influence of compact bone and other anatomic structures in tooth movement, specifically the distalization of maxillary teeth, and underline the associated inferences on mini-implant anchorage and the regional acceleratory phenomenon (RAP), which is reportedly enhanced by decortication or microperforation of the compact bone.
Boundaries of tooth movement: Primary role of compact bone
Compact bone includes the outer cortex of the alveolar process and the lining or “protective” shell of the socket, defined as the cribriform plate (anatomically) or lamina dura (radiographically) ( Fig 1 ). The multiperforation of this plate decreases its resistance to tooth movement compared with the outer and interdental compact bone. The bony elements are subject to inordinate individual variation in density, stiffness, and thickness. The interaction between these variables and orthodontic tooth movement is often limited to recording the rate of tooth movement relative to force systems.

To simulate the clinical settings without invading live tissues, researchers have resorted to finite element analysis (FEA), an engineering tool used to evaluate the response of structures to subjected loads by breaking down the entire structure into small elements. Orthodontic applications of FEA have consisted of testing a particular 3-dimensional (3D) model under various force loads to determine stress distribution by orthodontic appliances, however, not accounting for individual variation that reflects the array of clinical responses to the same mechanical setup. Indeed, the distal movement of a canine in the extracted space of a premolar may be slower or faster in different patients under similar force amount and direction.
To this end, we factored individual variation in a previous FEA study of the influence of cortical bone stiffness and thickness on distalization of maxillary posterior teeth against mini-implants (MIs) through direct anchorage (pull from MI to teeth) and indirect anchorage (teeth pulled against other teeth anchored by the MI). In a computed tomography-generated 3D model of the maxilla, the cortical bone was divided into areas of different material properties in the buccal and palatal regions defined in human cadavers, thus incorporating human variations in the model ( Fig 2 , A ). Increased stress values at the cortical bone adjacent to the molars indicated resistance to movement, particularly in the indirect modality (Fig. 2). Periodontal ligament stresses at the root surfaces were significantly different between adjacent teeth on stiffness variation but not with thickness variation, suggesting that cortical bone thickness did not influence initial displacement as much as stiffness.


This finding further denotes the existence of a minimal thickness necessary to offer resistance to tooth movement, beyond which stiffness is not affected. Average compact bone thickness around the posterior maxillary teeth ranges from 1.3 mm to 2 mm, with standard deviations within 0.5 mm. , Accordingly, a key element in tooth movement is the proximity of roots to a “shell” of compact bone. The additional thickness would not be more consequential because a thin but stiff layer of bone offers enough resistance to tooth movement.
Compact bone identification (“ID”)
At times advocated to support anchorage, compact bone can hinder tooth movement when edging the moved teeth, and cause side effects such as root resorption. The root-compact bone interface could be defined for personalized treatment planning by generating a patient’s compact bone identification including bone location and proximity to teeth to help determine the appropriate way to avoid its resistance to tooth movement. Individual bone topography can be mapped through cone-beam computed tomography imaging (if indicated), relating the stiffness of trabecular and cortical bones to their density, which has been reported to vary significantly on computed tomography scans of the interradicular areas of dentate maxillae. ,
Approaches to treatment cannot be generic because variable morphologic characteristics would discriminate low, medium, or high resistance to movement. Under low resistance, sequential (molar followed by other teeth) or en-masse distalization would succeed, whereas under high resistance, sequential distalization would better help overcome the anatomic barriers ( Fig 3 ).
Ideally, the teeth adjacent to compact bone should be steered away from the compact shell, a long-time premise often overlooked. However, moving the molars away from the surrounding cortex may be problematic if the alveolar bucco-palatal width is narrow, necessitating that tooth movement overcome the compact bone proper ( Fig 4 ). If measures to facilitate molar distalization such as the extraction of third molars or shifting to indirect anchorage are not successful, treatment alternatives should be considered (eg, extraction of premolar). Suggested clinical approaches when facing failure to molar distalization against MIs are shown in the Table .
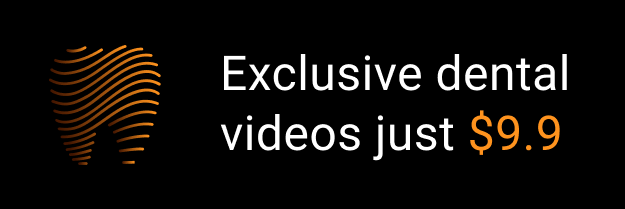