Abstract
Objectives
Contemporary adhesives lose their bond strength to dentin regardless of the bonding system used. This loss relates to the hydrolysis of collagen matrix of the hybrid layers. The preservation of the collagen matrix integrity is a key issue in the attempts to improve the dentin bonding durability.
Methods
Dentin contains collagenolytic enzymes, matrix metalloproteinases (MMPs) and cysteine cathepsins, which are responsible for the hydrolytic degradation of collagen matrix in the bonded interface.
Results
The identities, roles and function of collagenolytic enzymes in mineralized dentin has been gathered only within last 15 years, but they have already been demonstrated to have an important role in dental hard tissue pathologies, including the degradation of the hybrid layer. Identifying responsible enzymes facilitates the development of new, more efficient methods to improve the stability of dentin–adhesive bond and durability of bond strength.
Significance
Understanding the nature and role of proteolytic degradation of dentin–adhesive interfaces has improved immensely and has practically grown to a scientific field of its own within only 10 years, holding excellent promise that stable resin–dentin bonds will be routinely available in a daily clinical setting already in a near future.
1
Introduction
Since the first study to demonstrate the rapid time-related loss of dentin bond strength , numerous studies have confirmed the finding both with etch-and-rinse (E&R) and SE (SE) adhesives . The problem is specific for the resin–dentin bonds, as the resin–enamel bonds are very stable over time , and relates to the loss of the hybrid layer collagen matrix .
Enzymatic degradation of the collagen matrix by host-derived enzymes plays a significant role in the destruction of the bonded interface . To date, several matrix metalloproteinases (MMPs) and cysteine cathepsins have been identified in dentin, and suggested to be responsible for the digestion of collagen fibrils exposed at the adhesive interface. During the last 10 years, the understanding of the mechanisms involved in the proteolytic degradation of dentin–adhesive interfaces has gained immense attention and has practically grown to a scientific field of its own. The progression in this field has been rapid, and the clinical interest in measures to improve dentin bond durability is also increasing . This has led to several tentative approaches to prevent such enzymatic activity. In general, the strategy to improve the durability of resin–dentin bonds relies on the ability to inhibit the activity of the enzymes. In this review, we will look at the presence, identity and function of the collagen-degrading proteolytic enzymes in dentin.
2
Dentin collagen: structural properties vs. bonding
Before going into details of dentinal enzymes, it is essential to briefly overview the dentin organic matrix to fully understand the complexity of the tissue in relation to the adhesion and function of the enzymes. Approximately 50 vol.% of dentin is composed of minerals, the rest being type I collagen and non-collagenous proteins (30 vol.%) and water (approx. 20 vol.%) . During the mineralization of dentin and bone, collagen fibrils provide the aqueous compartment in which nanometer-sized apatite mineral crystals grow. In soft tissues (and in dentin and bone matrix prior mineralization), collagen intermolecular spaces contain highly ordered and tightly bound water molecules forming multi-layered cylinders around collagen molecules ( Fig. 1 ). During the mineralization process, water within and between the fibrils is progressively replaced with minerals . In mineralized dentin, there is no resin infiltration. To permit resin infiltration for adhesive retention, dental hard tissues are acid-etched by acids or acidic monomers to remove minerals that are replaced by rinse-water (E&R adhesives) or water used in SE primer-adhesives as a solvent (E&R and SE adhesives). Hydration of collagen prior to the monomer penetration is essential in order to avoid the matrix collapse due to the formation of interpeptide hydrogen bonds between collagen fibrils . In addition, the ionizable moieties of acidic monomers in SE adhesives are also hydrophilic and promote water sorption over time.
During application of adhesives, solvated comonomers are expected to replace the water and penetrate into and around collagen fibrils for proper hybridization . While the interfibrillar spaces between collagen fibrils in hybrid layers are wide enough to allow small hydrophilic monomers (e.g. HEMA, TEGDMA) to penetrate between the fibrils, intrafibrillar penetration has been challenged. The inter-molecular distance in the lateral packing of collagen molecules within a collagen fibril (the space supposed to be occupied by monomer molecules) is within range of 1.26–1.33 nm. Since even the adhesive monomers (such as TEGDMA) units are approximately 2 nm in diameter, the complete infiltration of the adhesive material even at single monomer molecular level is restricted by the space available within a collagen fibril . We will address this issue later in the article.
As adhesive comonomers diffuse into the water around collagen fibrils, their relatively high chemical concentrations (2–4 mol/L) lower the vapor pressure of water (Raoult’s law) making it more difficult to evaporate residual water using a dental air syringe . The spaces between collagen fibrils are between 20 and 30 nm. These spaces contain proteoglycan hydrogels , that may restrict the free diffusion of some of the larger adhesive monomers, such as BisGMA (512 Da) compared to small monomers such as HEMA (100 Da). Fig. 2 shows a schematic of collagen fibrils cut in cross-section (large circles) in a hybrid layer being infiltrated by a mixture of BisGMA/HEMA comonomers. Note that the small black dots, representing HEMA, diffuse more deeply than the large black dots representing BisGMA. This schematic was made to illustrate what might be responsible for a decreasing concentration of BisGMA that had been reported by Spencer and Wang when they examined its distribution across hybrid layers by micro-Raman spectroscopy.
However, as each collagen fibril is made up of hundreds of collagen molecules that are closely packed together, Bertassoni et al. argued that there is not sufficient space between packed collagen molecules (ca. 1.26–1.33 nm) to accommodate adhesive monomers ( Fig. 2 ).
Physiological experiments often provide insight into how molecules interact with collagen fibrils. Toroian et al. pulverized bovine bone into small particles and packed them into 2 cm × 50 cm columns to permit gel filtration column chromatography of these particles before and after demineralization. They used bone collagen particles just like investigators usually use hydrated Sephadex beads. In typical gel filtration chromatography, large molecular weight molecules like blue dextran (10 6 Da) are too large to penetrate the Sephadex beads, so they elute with the void volume ahead of molecules that can penetrate the beads and hence, whose elution is delayed. By using phosphate ions, glucose, osteocalcin (5700 Da), cytochrome (12.3 kDa), fetuin (48 kDa), hemoglobin (64 kDa) and IgG (152 kDa), Toroian et al. found that molecules less than 6 kDa easily diffuse between collagen molecules and entered “collagen water”, while molecules larger than 40 kDa were excluded from “collagen water”. However, they found that osteocalcin (5.7 kDa, molecular diameter 1.8 nm) did penetrate into “collagen water” even though X-ray diffraction studies confirmed that the Bragg spacing between hydrated collagen molecules is 1.8 nm ( Fig. 2 ). Generally, molecules whose diameters are equal to pore sizes in barriers are not permeable because the molecule would have to fit the pore perfectly. If they touched any part of the pore, they would be reflected away and not permeate.
Toroian et al. conclude that hydrated collagen molecules function as if their intermolecular size is significantly larger than 1.8 nm due molecular vibration/rotation. The results support the hypothesis “that individual collagen molecules have substantial freedom to move in the lateral plane of the fibril”.
We conclude that if osteocalcin (MW 5700 Da) can equilibrate with collagen water, then BisGMA (512 Da) and HEMA (100 Da) should easily permeate into water located around and within collagen molecules. Whether water-immiscible monomers such as BisGMA can wet collagen molecules is debatable. Since BisGMA is usually solvated in ethanol or HEMA, and they are both water miscible, perhaps dimethacrylates like BisGMA can infiltrate the space between collagen molecules and polymerize to envelop them and prevent protease-induced collagen hydrolysis.
Complete coverage of the nanoscale irregularities on the collagen fibrils surface via passive monomer penetration may be difficult to achieve. These irregularities are caused by the 4–6 nm height difference between the fibril gap and overlap zones, and nanometer-scale microfibrils at the surface of dentin collagen fibrils . Microfibrils, detected with X-ray diffraction, are discontinuous 5-mer repeats of collagen molecules with 4–5 nm diameters . Another potential hindrance may be proteoglycans (PGs), which with their glycosaminoglycan (GAG) carbohydrates envelop the collagen surface, retaining water . The ability of polymeric resins to fully displace water from the crevices present on collagen fibril surfaces may require extremely low viscosity monomeric material and precise placement of the primer components to alter the surface energy of the fibril. Nanoleakage can occur in the absence of detectable gaps in the resin–dentin interface . These “nanovoids” can be responsible for facilitating the diffusion of water molecules with diameters of about 100 pm, from the hydrated dentin , allowing slow but continuous degradation of the ester bonds in adhesive polymers. Degradation of the microfibrils would lead to nanoleakage, and with increasing void size, increase in dentinal fluid seepage would speed up the hydrolysis of both resins and matrix components, with eventual loss of bond strength. Others have argued that the ability of adhesive monomers to displace water from collagen is limited by their relative concentrations. Water has a concentration of 55 mol/L, while comonomers have concentrations of 3–4 mol/L . Thus, although the adhesive monomer HEMA has been shown to “fit” into the groove on water-free collagen peptides in computer models , there are structural and chemical reasons why this may not occur.
Other components and features of dentin matrix may also have marked impacts on the durability of dentin bonds. These include, e.g. collagen cross-linking and PGs in dentin. For the sake of clarity, these aspects will be described in detail in further paragraphs.
2
Dentin collagen: structural properties vs. bonding
Before going into details of dentinal enzymes, it is essential to briefly overview the dentin organic matrix to fully understand the complexity of the tissue in relation to the adhesion and function of the enzymes. Approximately 50 vol.% of dentin is composed of minerals, the rest being type I collagen and non-collagenous proteins (30 vol.%) and water (approx. 20 vol.%) . During the mineralization of dentin and bone, collagen fibrils provide the aqueous compartment in which nanometer-sized apatite mineral crystals grow. In soft tissues (and in dentin and bone matrix prior mineralization), collagen intermolecular spaces contain highly ordered and tightly bound water molecules forming multi-layered cylinders around collagen molecules ( Fig. 1 ). During the mineralization process, water within and between the fibrils is progressively replaced with minerals . In mineralized dentin, there is no resin infiltration. To permit resin infiltration for adhesive retention, dental hard tissues are acid-etched by acids or acidic monomers to remove minerals that are replaced by rinse-water (E&R adhesives) or water used in SE primer-adhesives as a solvent (E&R and SE adhesives). Hydration of collagen prior to the monomer penetration is essential in order to avoid the matrix collapse due to the formation of interpeptide hydrogen bonds between collagen fibrils . In addition, the ionizable moieties of acidic monomers in SE adhesives are also hydrophilic and promote water sorption over time.
During application of adhesives, solvated comonomers are expected to replace the water and penetrate into and around collagen fibrils for proper hybridization . While the interfibrillar spaces between collagen fibrils in hybrid layers are wide enough to allow small hydrophilic monomers (e.g. HEMA, TEGDMA) to penetrate between the fibrils, intrafibrillar penetration has been challenged. The inter-molecular distance in the lateral packing of collagen molecules within a collagen fibril (the space supposed to be occupied by monomer molecules) is within range of 1.26–1.33 nm. Since even the adhesive monomers (such as TEGDMA) units are approximately 2 nm in diameter, the complete infiltration of the adhesive material even at single monomer molecular level is restricted by the space available within a collagen fibril . We will address this issue later in the article.
As adhesive comonomers diffuse into the water around collagen fibrils, their relatively high chemical concentrations (2–4 mol/L) lower the vapor pressure of water (Raoult’s law) making it more difficult to evaporate residual water using a dental air syringe . The spaces between collagen fibrils are between 20 and 30 nm. These spaces contain proteoglycan hydrogels , that may restrict the free diffusion of some of the larger adhesive monomers, such as BisGMA (512 Da) compared to small monomers such as HEMA (100 Da). Fig. 2 shows a schematic of collagen fibrils cut in cross-section (large circles) in a hybrid layer being infiltrated by a mixture of BisGMA/HEMA comonomers. Note that the small black dots, representing HEMA, diffuse more deeply than the large black dots representing BisGMA. This schematic was made to illustrate what might be responsible for a decreasing concentration of BisGMA that had been reported by Spencer and Wang when they examined its distribution across hybrid layers by micro-Raman spectroscopy.
However, as each collagen fibril is made up of hundreds of collagen molecules that are closely packed together, Bertassoni et al. argued that there is not sufficient space between packed collagen molecules (ca. 1.26–1.33 nm) to accommodate adhesive monomers ( Fig. 2 ).
Physiological experiments often provide insight into how molecules interact with collagen fibrils. Toroian et al. pulverized bovine bone into small particles and packed them into 2 cm × 50 cm columns to permit gel filtration column chromatography of these particles before and after demineralization. They used bone collagen particles just like investigators usually use hydrated Sephadex beads. In typical gel filtration chromatography, large molecular weight molecules like blue dextran (10 6 Da) are too large to penetrate the Sephadex beads, so they elute with the void volume ahead of molecules that can penetrate the beads and hence, whose elution is delayed. By using phosphate ions, glucose, osteocalcin (5700 Da), cytochrome (12.3 kDa), fetuin (48 kDa), hemoglobin (64 kDa) and IgG (152 kDa), Toroian et al. found that molecules less than 6 kDa easily diffuse between collagen molecules and entered “collagen water”, while molecules larger than 40 kDa were excluded from “collagen water”. However, they found that osteocalcin (5.7 kDa, molecular diameter 1.8 nm) did penetrate into “collagen water” even though X-ray diffraction studies confirmed that the Bragg spacing between hydrated collagen molecules is 1.8 nm ( Fig. 2 ). Generally, molecules whose diameters are equal to pore sizes in barriers are not permeable because the molecule would have to fit the pore perfectly. If they touched any part of the pore, they would be reflected away and not permeate.
Toroian et al. conclude that hydrated collagen molecules function as if their intermolecular size is significantly larger than 1.8 nm due molecular vibration/rotation. The results support the hypothesis “that individual collagen molecules have substantial freedom to move in the lateral plane of the fibril”.
We conclude that if osteocalcin (MW 5700 Da) can equilibrate with collagen water, then BisGMA (512 Da) and HEMA (100 Da) should easily permeate into water located around and within collagen molecules. Whether water-immiscible monomers such as BisGMA can wet collagen molecules is debatable. Since BisGMA is usually solvated in ethanol or HEMA, and they are both water miscible, perhaps dimethacrylates like BisGMA can infiltrate the space between collagen molecules and polymerize to envelop them and prevent protease-induced collagen hydrolysis.
Complete coverage of the nanoscale irregularities on the collagen fibrils surface via passive monomer penetration may be difficult to achieve. These irregularities are caused by the 4–6 nm height difference between the fibril gap and overlap zones, and nanometer-scale microfibrils at the surface of dentin collagen fibrils . Microfibrils, detected with X-ray diffraction, are discontinuous 5-mer repeats of collagen molecules with 4–5 nm diameters . Another potential hindrance may be proteoglycans (PGs), which with their glycosaminoglycan (GAG) carbohydrates envelop the collagen surface, retaining water . The ability of polymeric resins to fully displace water from the crevices present on collagen fibril surfaces may require extremely low viscosity monomeric material and precise placement of the primer components to alter the surface energy of the fibril. Nanoleakage can occur in the absence of detectable gaps in the resin–dentin interface . These “nanovoids” can be responsible for facilitating the diffusion of water molecules with diameters of about 100 pm, from the hydrated dentin , allowing slow but continuous degradation of the ester bonds in adhesive polymers. Degradation of the microfibrils would lead to nanoleakage, and with increasing void size, increase in dentinal fluid seepage would speed up the hydrolysis of both resins and matrix components, with eventual loss of bond strength. Others have argued that the ability of adhesive monomers to displace water from collagen is limited by their relative concentrations. Water has a concentration of 55 mol/L, while comonomers have concentrations of 3–4 mol/L . Thus, although the adhesive monomer HEMA has been shown to “fit” into the groove on water-free collagen peptides in computer models , there are structural and chemical reasons why this may not occur.
Other components and features of dentin matrix may also have marked impacts on the durability of dentin bonds. These include, e.g. collagen cross-linking and PGs in dentin. For the sake of clarity, these aspects will be described in detail in further paragraphs.
3
Enzymes in dentin
3.1
Matrix metalloproteinases
Matrix metalloproteinases (MMPs) are a family of Zn 2+ – and Ca 2+ -dependent enzymes, that in concert are able to degrade practically all ECM components, making them important components in many biological and pathological processes. Esterases and proteases are also classified as hydrolases. That is, they enzymatically add water across ester or peptide bonds. In humans, the MMP family contains 23 members that are frequently divided into six groups – collagenases, gelatinases, stromelysins, matrilysins, membrane-type MMPs (MT-MMPs), and other MMPs – based on substrate specificity and homology . Even though this classification is commonly used both for historical and for practical reasons, it does not fully reflect the complexicity of MMP functions and biological activities, as most MMPs can degrade several substrates with variable specificity . For example, collagenases-1 and -3 (MMP-1 and -13) can also degrade gelatin at a slow rate, and gelatinases (MMP-2 and -9) can degrade several types of collagen, especially type IV collagen (thus the old name “type IV collagenases”) . Apart from their ECM degradation, MMPs also play important roles in the conversion of noncollagenous matrix proteins to signaling molecules that affect cell survival, proliferation, and differentiation .
MMPs are synthesized and mostly secreted as inactive proenzymes (zymogens), in which the so-called prodomain is present, inhibiting the functional activity of catalytic domain ( Fig. 3 ). In latent (non-activated) MMPs, the unpaired cysteine in the prodomain forms a bridge with the catalytic zinc (referred to as the “cysteine switch” mechanism ), preventing enzymatic activity. This conserved cysteine acts as a ligand for the catalytic zinc atom in the active site, excluding water molecules and rendering the enzyme inactive. Activation occurs when this linkage is disrupted by proteolytic cleavage of the propeptide by other MMPs, cysteine cathepsins or other proteinases, or chemically, e.g. by amino phenyl mercuric acid (APMA), replacing the thiol group with water . In addition to the pro- and catalytic domains, MMPs contain other domains responsible, for instance, for substrate specificity, recognition, and interaction . Therefore, MMPs are often classified according to their molecular structures .
Collagen molecules contain a rigid helical center ( Fig. 4 ) with globular N- and C-terminal ends called telopeptides. In vertebrate MMP family, collagenases (MMP-1, -8, -13), MMP-2 (gelatinase A), and membrane-type 1-MMP (MT1-MMP, MMP-14) can cleave native triple-helical type I, II, and III collagens. They all cleave collagens into ¼- and ¾-fragments at the Gly-Leu/Isoleu peptide bond where collagen peptide structure determines both specific cleavage and binding sites for MMPs . The fragments denature at body temperature, and are further degraded by gelatinases and other non-specific tissue proteinases. Interstitial collagen consists of three α chains, and each chain winds around each other in a right-handed twist to form a triple helical structure with about 300 nm in length and 1.5 nm in diameter. This triple-helical structure makes interstitial collagens fibrils in their native configuration resistant to most vertebrate proteolytic enzymes. The substrate-binding site of collagenases is located in a deep cleft with the entrance being only approximately 0.5 nm wide. This is not enough space to accommodate triple helical collagen with a diameter that is 3 times larger than the active site of the enzyme . Currently, two mechanisms have been proposed to allow the degradation of collagen fibril by MMPs. Using a MMP-1 mutant that is unable to cleave collagen but retains the binding properties, Chung et al. demonstrated that MMP-1 is capable of unwinding collagen fibril to allow the enzyme’s active site to cleave individual chains in succession ( Fig. 4 ). The role of MMP in unwinding collagen peptide helices was further supported by the cleavage of unwound fibril by MMP-3 and neutrophil elastase, which normally do not degrade fibrillar collagen . The similar binding-unwinding mechanisms have later been suggested for MMP-8, -2 and -14 . This view was at least partially challenged by Perumal and others , when they demonstrated that the collagen region where cleavage begins, is fully protected by the collagen C-telopeptide (the terminal end of the molecule) restricting the access of MMPs to the cleavage site of the native collagen structure . They suggest that the site must be exposed by proteolytical removal of C-terminal telopetide by telopeptidase or, e.g. mechanical damage, before MMP can bind to the substrate’s “interaction domain” facilitating the triple-helix unwinding/dissociation function of the enzyme before collagenolysis. Alternatively, damage caused, e.g. by physical loading may induce changes in fibril structure, such as breakage of cross-linkages at the C-terminus. This damage may expose the first collagen peptide chain to be cleaved by MMP, leading to a greater freedom of movement for other chains and their subsequent cleavage . Collagen molecules distal to the original cleavage site would also become accessible to MMP cleavage, leading to further degradation . This is an inviting hypothesis, as the damages caused to dentin collagenous matrix by demineralization in caries lesions, phosphoric acid or acidic monomers have been suggested to cause changes in the collagen molecular arrangement (e.g. breakage of cross-linkages) which may expose the catalytic binding site . Interestingly, telopeptidase enzyme activity of MMP-9 has long been suggested to be important in bone matrix degradation . MMP-9 is present in dentin , it is readily activated by acid pH changes , and telopeptidase activity of MMP-9 has been suggested to have a role in dentin matrix degradation in caries . Alternatively, cysteine cathepsins may be involved. Cysteine cathepsins can activate MMPs , and this mechanism has been suggested to be involved in human dentinal caries lesions . Cysteine cathepsins can also cleave C-terminal telopeptide of type I collagen , possibly exposing the collagenase cleavage site after being activated by acids.
Although intermolecular cross-links can occur along both helical and telopeptide regions, they are especially common in the telopeptide domains. When Garnero et al. incubated acid-etched bone powder with pure, soluble MMP-2, they saw increased liberation of soluble collagen peptides. When the peptides were isolated by SDS-PAGE electrophoresis, none of the bands were due to ¼ or ¾ length collagen fragments. Using specific antibodies directed at various cross-linked telopeptides, they found that MMP-2 released relatively long cross-linked C-terminal telopeptide fragments called ICTP fragments. When they incubated bone powder with cathepsin K, the released telopeptides were much shorter. These were called CTX peptides. Both classes of peptides are now measured in plasma and urine in postmenopausal patients as indices of the degree of bone turnover.
When Osorio et al. incubated exogenous soluble MMP-2 with completely demineralized beams of dentin, the dentin released ICTP telopeptide fragments. The control beams incubated in control media without MMP-2 also released ICTP peptide fragments, albeit at lower concentrations, indicating that the MMP-2 isolated from dentin may function as a “telopeptidase”. If gelatinases MMP-2 and -9 can hydrolyze the bulky telopeptides off surface collagen fibrils, they may create enough space for a collagenase to bind to the proper binding site to create unwinding of the collagen molecule that promote collagen hydrolysis. It appears that MMPs may work in concert. Gelatinases may indeed hydrolyze gelatin when it forms, but may also help initiate collagenase activity by being a “telopeptidase”.
MMP activity can be regulated at multiple levels, e.g. transcription, secretion, degranulation of enzymes from intracellular granules, and by specific and non-specific inhibitors . Tissue inhibitors of MMPs (TIMP-1 to TIMP-4) are specific inhibitors that participate in controlling the local activities of MMPs in tissues. Most TIMPs inhibit active MMPs and some TIMPs can prevent pro-MMP activation. TIMPs are also involved in various cellular and tissue regulatory processes . In body fluids, a macroglobulin protein, α2-macroglobulin, may be the primary regulator of MMP activity. α2-macroglobulin and fetuin-A (aka, α-2-Heremans Schmid-glycoprotein, α-2-HS-glycoprotein, AHSG), are abundant plasma proteins that may be involved in the regulation of dentin MMPs . There are also an ever-increasing number of synthetic MMP inhibitors. Most of them prevent MMP activity by chelating or replacing the active site Zn 2+ , or by “coating” the substrate.
Several MMPs have been identified in the dentin–pulp complex. During tooth development, MMPs participate in the organization of the ECM components and compartments . Mature human odontoblasts synthesize at least gelatinases MMP-2 and -9, collagenases MMP-8 and -13, and enamelysin MMP-20 . However, the gene expression profile is much larger, covering most of the MMP family members .
The first time collagenase in dentin was described was in the early 1980s, when Dayan et al. demonstrated collagenolytic activity in carious and intact human dentin. The finding went by pretty much unexplained and unnoticed, which is not surprising, since at that time MMP research was still in its “adolescence” phase, as described by Lapière . For example, the name “matrix metalloproteinases” for the family was only suggested in 1986 . Recent studies have demonstrated the presence of at least gelatinases MMP-2 and -9, collagenase MMP-8 and stromelysin MMP-3 in human dentin . MMP-20 is present in dentinal fluid , intense gelatinolytic activity has been observed in dentinal tubules with laser confocal microscopy ( Fig. 5 ), and MMP-20 , -2 and -9 have been shown to increase in dentinal tubules of carious teeth. In mineralized dentin, MMP-2 is probably the most abundant MMP . TIMP-1 and -2 are also found in human dentin ; α2-macroglobulin and fetuin-A are also present in marked quantities in dentin . The physiological roles of MMPs in dentin is not well understood, but they have been suggested to participate in peritubular and tertiary dentin formation, and in the release of dentinal growth factors . The role of MMPs in collagen degradation in dentin caries progression has been well documented . For a comprehensive overview of current knowledge of MMPs in dentin–pulp complex, the reader is referred to recent reviews .
3.2
Cysteine cathepsins
The lysosomal cysteine proteases belong to the clan CA of cysteine proteases; they are members of the C1 family of papain-like enzymes, the largest and the best-characterized family of cysteine peptidases. There are 11 human cysteine cathepsins, namely cathepsins B, C, F, H, K, L, O, S, V, X and W . Cathepsins B, H, L, C, X, F, O and V are ubiquitously expressed in human tissues, while cathepsins K, W and S are tissue-specific . They are biosynthesized in the secretory route as inactive zymogens and are transported to endosomes via the mannose-6-phosphate receptor pathway . Cysteine cathepsin zymogens are processed to the active forms either autocatalytically triggered by endosomal acidification or by other proteases . The autocatalytic activation of cathepsins is substantially accelerated in the presence of anionic polysaccharides, such as GAGs and dextran sulfate . The structure–function is best known for cathepsin B .
Cysteine cathepsins are active and stable in a slightly acidic pH and mostly unstable at neutral pH. They can be irreversibly inactivated at neutral pH , except cathepsin S which is stable at neutral or slightly alkaline pH . Cathepsin B has mainly peptidyl-dipeptidase and carboxypeptidase activity . On the other hand, cathepsin B endopeptidase activity has a pH optimum around 7.4 , which is expectable for an enzyme that is involved in many physiological processes related with extracellular matrix degradation. Unlike other cysteine proteases, cathepsin B has a flexible loop that partially occludes the active site aperture of mature enzyme and carries pH-sensitive arginine and histidine residues, and appears to play crucial role in modulating its endo- and exopeptidase activities .
Cysteine proteases are often termed promiscuous proteases or those with broad substrate specificity . Physiological substrates for cathepsin B, and others, are still a matter of speculation. However, cathepsin B degrades extracellular matrix components such as type IV collagen and fibronectin under both acidic and neutral pH .
Although cysteine cathepsins were initially considered as intracellular enzymes responsible for the non-specific proteolysis in the endosomal/lysosomal compartment, this view is rapidly changing . In some (non-dental) cell types, the secretion processes of cathepsins are very well elucidated. Cathepsin B can be found in lysosomes or secretory vesicles , or may follow the default pathway of secretion . Furthermore, cathepsin B is consistently found associated with the plasma membrane of tumor cells . Although lysosomes were for a long time considered to be dead-end organelles, recent developments show that these organelles or their contents in shuttle vesicles can move back and forth along microtubules rendering possible exocytosis or retrograde transport to endosomes .
Several cathepsins participate in bone resorption and remodeling. The most potent mammalian collagenase is cathepsin K which, in contrast to the cathepsins B, L and S, is highly expressed in osteoclasts and odontoclasts , playing a special role in mineralized tissue resorption under normal and pathological conditions . Indeed, in the absence of cathepsin K, osteoclasts are hampered in their capacity to digest bone collagen . The biological relevance of the collagenolytic activity of cathepsin K was underlined by the finding that deficiency in this protease causes the bone-sclerosing dysplasia, pycnodysostosis, in man and an osteopetrotic phenotype in mice . When osteoclasts demineralize bone matrix by lowering the pH in the resorption lacuna, the acidic environment favors the extracellular collagenolytic activity of cysteine proteases, especially cathepsin K. Later, when the pH has increased due to an increased amount of phosphate and calcium, the MMPs digest the rest of collagen . Low pH around joint implants also leads to demineralization and type I collagen degradation in peri-implant bone; the acid- and cathepsin K-driven mechanisms of periprosthetic bone resorption have been described in context with loosening of hip implants .
Recent data have shown that odontoblasts and pulp tissue have coding DNA sequences (CDS) of most cathepsins , suggesting that, in terms of protein expression, the variety of cysteine cathepsins present in dentin–pulp complex can be compared to that described for MMPs . Cysteine cathepsin activity has been demonstrated both in intact and carious dentin, and cathepsin B has been localized in dentinal tubules . Once synthesized by odontoblast and/or pulp tissue, secreted cathepsins can easily reach the dentinal tubules and enter deep into dentin ( Fig. 6 ). Moreover, changes in expression levels, localization and activity have been described in carious dentin . The significant increase of cysteine cathepsin activity in carious dentin with increasing depth (approaching the pulp) indicates that odontoblast- or pulp-derived cysteine cathepsins may be important in active caries lesions, especially with young patients . Together these findings indicate that cysteine cathepsins may have an important role during dentinal caries development. Since clinical restorations are practically always made on carious teeth with at least some degree of pulpal inflammation, it is possible that increased levels of cysteine cathepsins are present also in dentinal tubules under the restoration.
The increased expression and/or activity of cysteine cathepsins often coincide with their presence in the extracellular environment, confirming the hypothesis that pH is not sole factor responsible for their activity. Cathepsins with high collagenolytic activities are known to be mainly responsible for remodeling the extracellular matrix . The extracellular matrix consists mostly of collagen and proteoglycans, which have all been identified as the cathepsin substrates . Cathepsin K is the only cysteine cathepsin capable of cleaving the collagen at the triple helical region . However, gelatin can be cleaved by several cathepsins and cathepsins B and L cleave in the non-helical telopeptide extensions of collagens . Cathepsins L and S can release GAGs from cartilage proteins such as aggrecan . PGs form interfibrillar bridges that absorb water with their negatively charged GAG side chains in dentin collagenous matrix , and GAGs play a crucial role in the formation of complexes between cysteine cathepsins and their protein substrates. This will be discussed in more detail in the next section.
3.3
The interplay of dentin proteolytic enzymes and non-collagenous proteins
Since the role of MMPs in dentin pathologies first emerged less than 15 years ago , and cysteine cathepsins were detected in dentin only 2 years ago , it is obvious that not much is known about their potential interactions in dentin formation, physiology, or diseases, let alone in the loss of collagen matrix in the hybrid layer. However, the exceptionally wide expression profile of MMPs and cysteine cathepsins in mature human odontoblasts strongly indicate that the enzymes must have various tasks in the formation and maturation of mineralized dentin. This kind of wide-scale expression of two extracellular matrix-degrading enzyme families in specific tissue is quite unique, especially the cells that in general are not considered to participate in tissue remodeling under physiologic conditions. Most of the potential interactions between MMPs and cysteine cathepsins in dentin are still based on the research with other tissues, and must be considered speculative until more work has been performed in dentin.
In cartilage collagen, cathepsin B and MMPs have been suggested to have at least partially independent pathways in cartilage proteoglycan breakdown . Cysteine cathepsins are able also to activate MMPs . Cathepsin B from articular chondrocytes increases MMP levels and stimulates angiogenesis by proteolytic inhibition of TIMPs . Moreover, procathepsin B can be activated by active MMPs , and conversely, cathepsin B has been shown to be responsible for activation of MMP-1 in gingival fibroblast cultures . This scenario establishes a possible and reasonable conclusion that both class of proteolytic enzymes, cysteine cathepsins and MMPs, are participating synergistically in bone resorption and make cathepsin B an attractive candidate for the potential cathepsin–MMP interplay also in dentin–pulp complex ( Fig. 7 A and B ). Identification of different cathepsins at the protein level in dentin may, in the future, better elucidate the potential cathepsin–MMP interactions also in the degradation of the hybrid layer collagen.
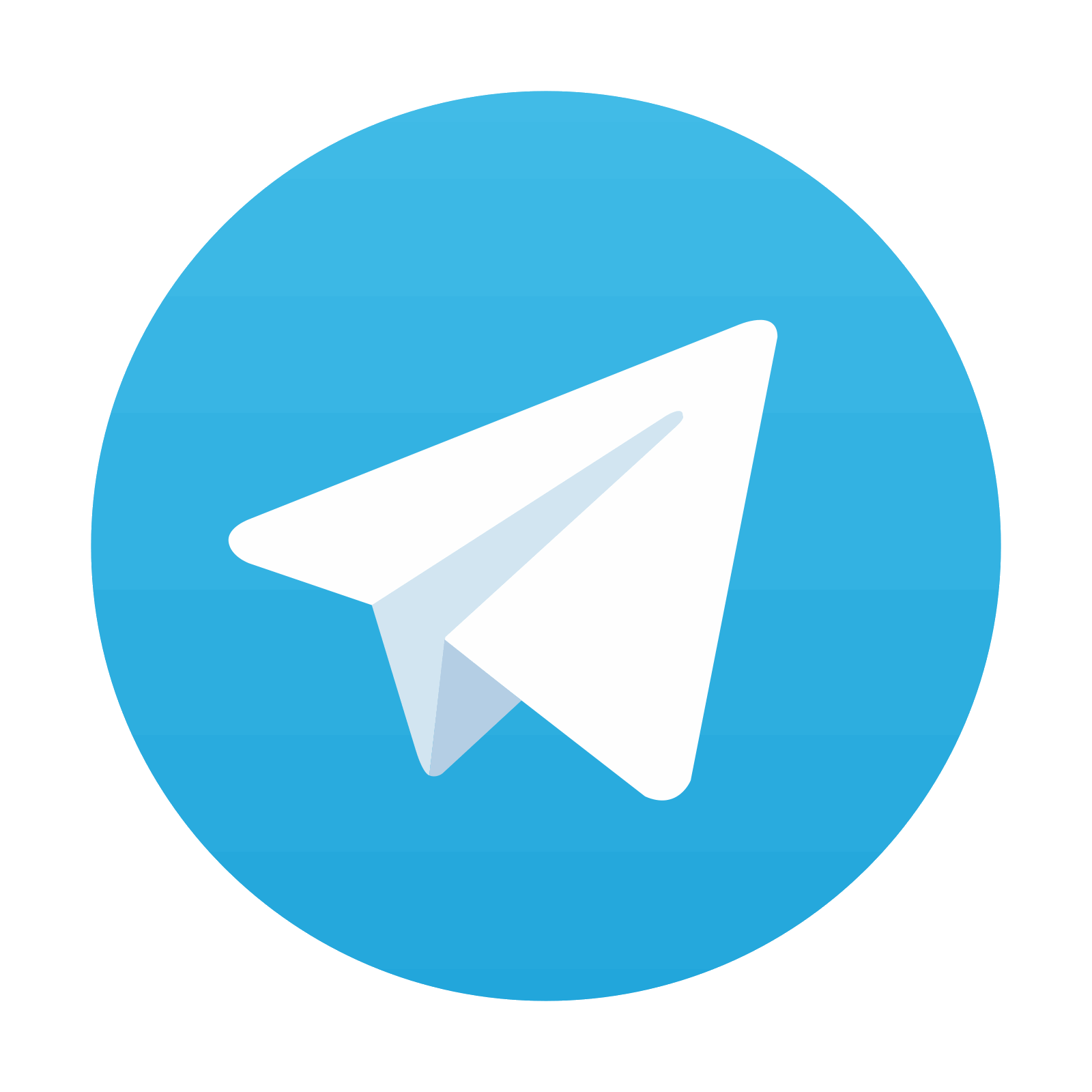
Stay updated, free dental videos. Join our Telegram channel

VIDEdental - Online dental courses
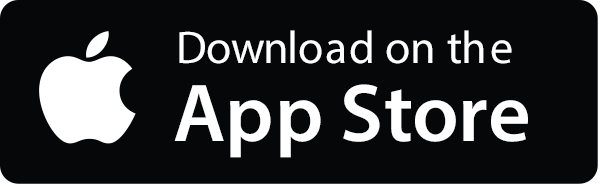
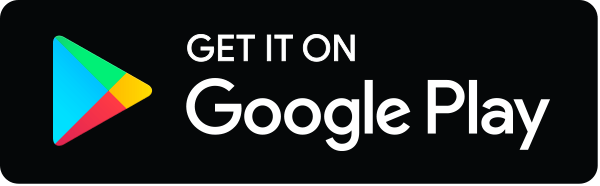
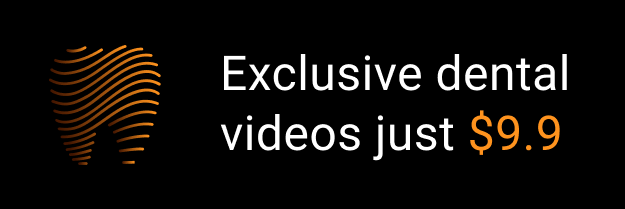