Highlights
- •
Infiltrating the zirconia cementation surface with glass can substantially improve its resin bonding strength.
- •
The interfacial fracture energy for glass-infiltrated graded zirconia is similar to that of porcelain.
- •
Current methods for determining the interfacial fracture energy G C of resin-ceramic bonds are marred by uneven stress distribution at the interface.
- •
A wedge-loaded double-cantilever-beam specimen is developed to accurately measure G C in adhesively bonded zirconia-based restorative materials.
Abstract
Objective
A major limiting factor for the widespread use of zirconia in prosthetic dentistry is its poor resin-cement bonding capabilities. We show that this deficiency can be overcome by infiltrating the zirconia cementation surface with glass. Current methods for assessing the fracture resistance of resin-ceramic bonds are marred by uneven stress distribution at the interface, which may result in erroneous interfacial fracture resistance values. We have applied a wedge-loaded double-cantilever-beam testing approach to accurately measure the interfacial fracture resistance of adhesively bonded zirconia-based restorative materials.
Methods
The interfacial fracture energy G C was determined for adhesively bonded zirconia, graded zirconia and feldspathic ceramic bars. The bonding surfaces were subjected to sandblasting or acid etching treatments. Baseline G C was measured for bonded specimens subjected to 7 days hydration at 37 °C. Long-term G C was determined for specimens exposed to 20,000 thermal cycles between 5 and 55 °C followed by 2-month aging at 37 °C in water. The test data were interpreted with the aid of a 2D finite element fracture analysis.
Results
The baseline and long-term G C for graded zirconia was 2–3 and 8 times greater than that for zirconia, respectively. More significantly, both the baseline and long-term G C of graded zirconia were similar to those for feldspathic ceramic.
Significance
The interfacial fracture energy of feldspathic ceramic and graded zirconia was controlled by the fracture energy of the resin cement while that of zirconia by the interface. G C for the graded zirconia was as large as for feldspathic ceramic, making it an attractive material for use in dentistry.
1
Introduction
In recent years, zirconia has emerged as a promising restorative material for dental crowns and fixed dental prostheses due to its superior mechanical properties and excellent biocompatibility. However, owing to its chemical inertness zirconia suffers from poor bonding to adhesive resin cements, which greatly limits its applications in adhesively bonded dental restorative prostheses such as Maryland bridges, cantilever bridges, partial crowns, inlays and onlays. Several surface treatment approaches for improving zirconia-resin bonding were offered which may be categorized as mechanical, chemical or a combination of both. Successful examples for mechanical treatments are sandblasting with alumina particles and porous nano-structured alumina coating , whereas those for chemical treatments include thin silica-based coatings and surface functionalization using various chemical treatments . Chemo-mechanical treatments have also been widely used for this purpose, including co-jet sandblasting and more recently surface glass-infiltration . It has been reported that modification of zirconia surface by glass-infiltration improves the esthetics of dental crowns , as well as flexural strength and resistance to veneer/core delamination yet without compromising key mechanical properties such as resistance to occlusal cracking and edge chipping . An important benefit of this approach in the present context is that it provides a glass rich surface that allows the application of standard etching-silane cementation techniques. The resin-cement bonding quality of the surface of the glass-infiltrated graded zirconia, the latter to be referred here as “graded zirconia”, has not been examined, however.
A number of testing approaches for evaluating bond strength in applications to restorative prosthetic materials have been suggested, the most commonly used in adhesive dentistry being the shear bond strength (SBS) and microtensile bond strength (MTBS) (e.g., ). Such methods have been used to evaluate the effect of surface treatment or thermocycling of ceramic restorative materials ; thermocycling has been used extensively to simulate aging of resin cement in an intra-oral environment . While relatively simple, such testing concepts are generally marred by large variations in the bond failure stress, the effect that may be attributed to the joint’s sensitivity to geometric misalignments and the tendency for tensile stresses to concentrate at the bond terminus . An alternative means for assessing bond strength is the use of fracture mechanics. In this approach, the bond includes an initial crack starter which facilitates a smooth crack initiation and growth thus eliminating the sensitivity to flaws and stress gradients and reducing the effect of geometric misalignments. The quantity of interest in this case is the fracture energy (per unit area) needed to extend the starting crack, G C , rather than the failure stress. A popular means for evaluating G C in applications to adhesively bonded joints is the double-cantilever-beam (DCB) specimen. In this case, the bond is subjected to tensile stress at the crack tip, which is generally associated with the most critical form of fracture . In addition to the relative ease in specimen fabrication and testing, the calculated fracture energy is little sensitive to the cement layer thickness , which may vary greatly in a clinical setting.
In this work, we examined the efficacy of surface glass-infiltration on the resin-cement bond properties of zirconia. The interfacial fracture energy was determined using the DCB specimen. Both short-term (7 days hydration at 37 °C) and long-term (20,000 thermal cycles between 5 and 55 °C followed by a 2-month aging at 37 °C) in vitro simulations were considered. A widely used commercial dental feldspathic ceramic was chosen as a reference bonding material.
2
Materials and methods
Fig. 1 shows the wedge double-cantilever-beam specimen (WDCB) used. Details of the fabrication and testing procedures follow.

2.1
Materials
Three ceramic restorative materials were tested: zirconia, graded zirconia and feldspathic ceramic. The zirconia (5.18 wt.% Y 2 O 3 , TZ-3Y-E grade, Tosoh, Tokyo, Japan) specimens were fabricated from cold isostatic pressed and lightly sintered tiles (14 × 35 × 80 mm 3 ). The tiles were cut into 3.8 × 3.8 × 35 mm 3 bars and sintered at 1450 °C for 2 h. The preparation of graded zirconia has been described earlier . Briefly, an in-house developed glass with composition similar to dental porcelain and CTE machting that of the zirconia (10.5 × 10 −6 °C) was prepared. The main composition of the glass by weight was 65.5% SiO 2 , 11.7% Al 2 O 3 , 10.0% K 2 O, 7.3% Na 2 O, 3.0% CaO. The glass, in the form of powder slurry, was first applied on pre-sintered Y-TZP bars (1350 °C for 1 h). Glass-infiltration and densification occurred in a single process at 1450 °C for 2 h. The resulting structure consisted of a 15–40 μm thick residual glass layer followed by a 120 μm thick graded layer where the content of intergranular glass gradually diminishes and finally transitions into a dense Y-TZP interior. All specimen surfaces except for the cementation ones were ground and polished down to 6 μm grade finish, resulting in the following final dimensions for the zirconia and graded zirconia: ( b , h , L ) = (3, 3, 30) mm 3 where, as shown in Fig. 1 , b , h , and L are the depth, thickness and length of the specimen bars, in that order. The feldspathic ceramic bars were cut from commercial large CAD/CAM blocks (VITABLOCS TriLuxe forte, a fine-structure feldspar ceramic). As for zirconia, all surfaces except for the cementation ones were polished down to 6 μm grade finish. The specimen final dimensions for feldspathic ceramic were ( b , h , L ) = (3, 5, 30) mm 3 . To guide the insertion of a crack opening wedge, prior to bonding the top edges of the cementation surfaces of all bar specimens were beveled at 45° relative to the long axis of the beam ( Fig. 1 ) by means of grinding using 10 μm diamond impregnated polishing discs under water cooling. The vertical length of the beveled region was set at 1 mm. Since the bond-normal ( F x ) and compressive ( F y ) load components transmitted to the specimen edge are unaffected by this edge beveling, and the crack tip is at least 4 mm away from the beveled edges, the effect of material beveling on the measured G C value is deemed negligible. The cementation surface of zirconia and graded zirconia were kept in their sintered and infiltrated stage, respectively, whereas those of feldspathic ceramic were abraded with 15 μm diamond disk in preparation for further surface treatments, i.e. acid etching or sandblasting.
2.2
Cementation
One-hundred cemented specimens (5 Groups, n = 20) were prepared by adhesive cementation of two identical ceramic bars with a 10-methacryloyloxydecyl dihydrogen phosphate (MDP) containing dental cement (Panavia F 2.0, Kuraray, Osaka, Japan). The selection of this cement was motivated by a previous study showing that the MDP-containing composite resin cement Panavia F coupled with a sandblasted zirconia surface gives rise to a long-term durable bond . The cementation surfaces of the ceramic bars were subject to sandblasting or acid etching, commonly used practices for enhancing bond strength . The specimens in each of the five groups were categorized based on surface preparation and ceramic materials as follows: Groups 1, 2, and 3 – zirconia (Yttria-tetragonal zirconia polycrystals), graded zirconia, and feldspathic ceramic (VITABLOCS TriLuxe forte), in that order, each sandblasted with aluminum oxide (Al 2 O 3 ) particles; Group 4 – graded zirconia acid etched with 5% hydrofluoric acid (VITA Ceramic Etch) for 2 min; Group 5 – feldspathic ceramic acid etched with 5% hydrofluoric acid (VITA Ceramic Etch) for 1 min. The parameters for sandblasting were: 50 μm alumina particles at 1 bar for graded zirconia and feldspathic ceramic, and 2 bars for zirconia, 1 cm stand-off distance and 2 s/cm 2 .
After surface treatment, the cementation protocol followed manufacturer’s instructions for Panavia F 2.0. All bars were cleaned using distilled water in an ultrasonic bath (Solid state/ultrasonic T-14B, L&R, New Jersey, USA) for 2 min. A thin layer of K-etchant gel (Kuraray, Osaka, Japan) was applied to the cementation surface with a small micro-brush. After 5 s, the layer was washed thoroughly and the bars dried with oil- and moisture-free compressed air. Clearfil Ceramic Primer (Kuraray, Osaka, Japan), an MDP containing coupling agent, was applied to the prepared surface of the bar with a micro-brush and dispersed with oil- and moisture-free air stream for 5 s.
A 50 μm thick aluminum foil was placed at the end of the beveled edge of one of the two bonding bars to help control the thickness of cement between the bars. Equal amounts of paste A and Paste B of Panavia F 2.0 were mixed with a spatula for 20 s after which the mixture was applied to the bonding surface. The two bars were then pressed together for 30 s using a force of 9.8 N. After removing the aluminum foil, the bonding surfaces were light cured for 20 s (Ultra Lume LED 5, UT, US). The cemented bars were stored in distilled water at 37 °C in an incubator for a period of 7 days in order to hydrate the cement .
After curing the surface of the observed edge of the bond was polished with a 6 μm diamond suspension. The adhesive thickness, measured along the bonded interface using an optical microscope, typically varied from 30 to 80 μm. A cut ∼0.5 mm deep was created along the bond line, just below the beveled edges, using a 0.3 mm thick diamond impregnated wheel soaked in water coolant. The introduction of this narrow cut was to help start a smooth crack growth.
2.3
Thermocycling and storage
The specimens in each group (5 groups, n = 20) were subjected to 2 different storage conditions before testing: (1) 7 days incubation in distilled water at 37 °C (5 groups, n = 10); (2) 20,000 thermal cycles between 5 and 55 °C and 60 days incubation at 37 °C (5 groups, n = 10). The thermal cycle treatments were carried out by alternately submerging the bonded specimens between two water baths of 5 and 55 °C with a dwell time of 30 s at each temperature. Note that 20,000 cycles have been estimated to be equivalent to a period of 2 years in the oral cavity . To further simulate intraoral conditions, all thermocycled specimens were stored in the incubator for an additional 60 days at 37 °C .
2.4
Testing
Fig. 1 shows the specimen in the loading stage. The lower part of the specimens was firmly gripped by a vice over a distance e = 8 mm while the upper part pressed down by a hardened steel wedge of total angle 2 β = 60° at a constant displacement rate of 0.1 mm/min using a standard loading frame. The evolution of cracking was observed from the polished specimen edge using a video camera equipped with a telescopic lens (Optem, Inc.). To help locate the crack tip, a paper scale bar was glued along the bonded interface prior to loading. After unloading, the fracture surfaces were observed with an optical microscope or a scanning electron microscope (SEM). From the video footage and machine output the load P i to initiate rapid fracture and corresponding crack length c i were documented for each test.
2.5
Fracture energy calculation
The variation of energy release rate G (energy per unit area) with crack length c for the specimen shown in Fig. 1 was determined using a commercial FEM code (Ansys Inc.) specified to plane strain conditions. Because of its relative smallness, the adhesive thickness was neglected in the calculations. From dimensional consideration the energy release rate G takes the form (e.g., )
where P is the applied load, E is the Young’s modulus of the bar, and f is some function of c / h . Assuming, for simplicity, that the load from the wedge is transmitted to the specimen arms by frictionless contact, it can be easily shown that the horizontal and vertical components of the applied load per unit specimen width, F x and F y , respectively, are given by:
The energy release rate in the specimen was determined using Irwin’s crack opening displacement approach:
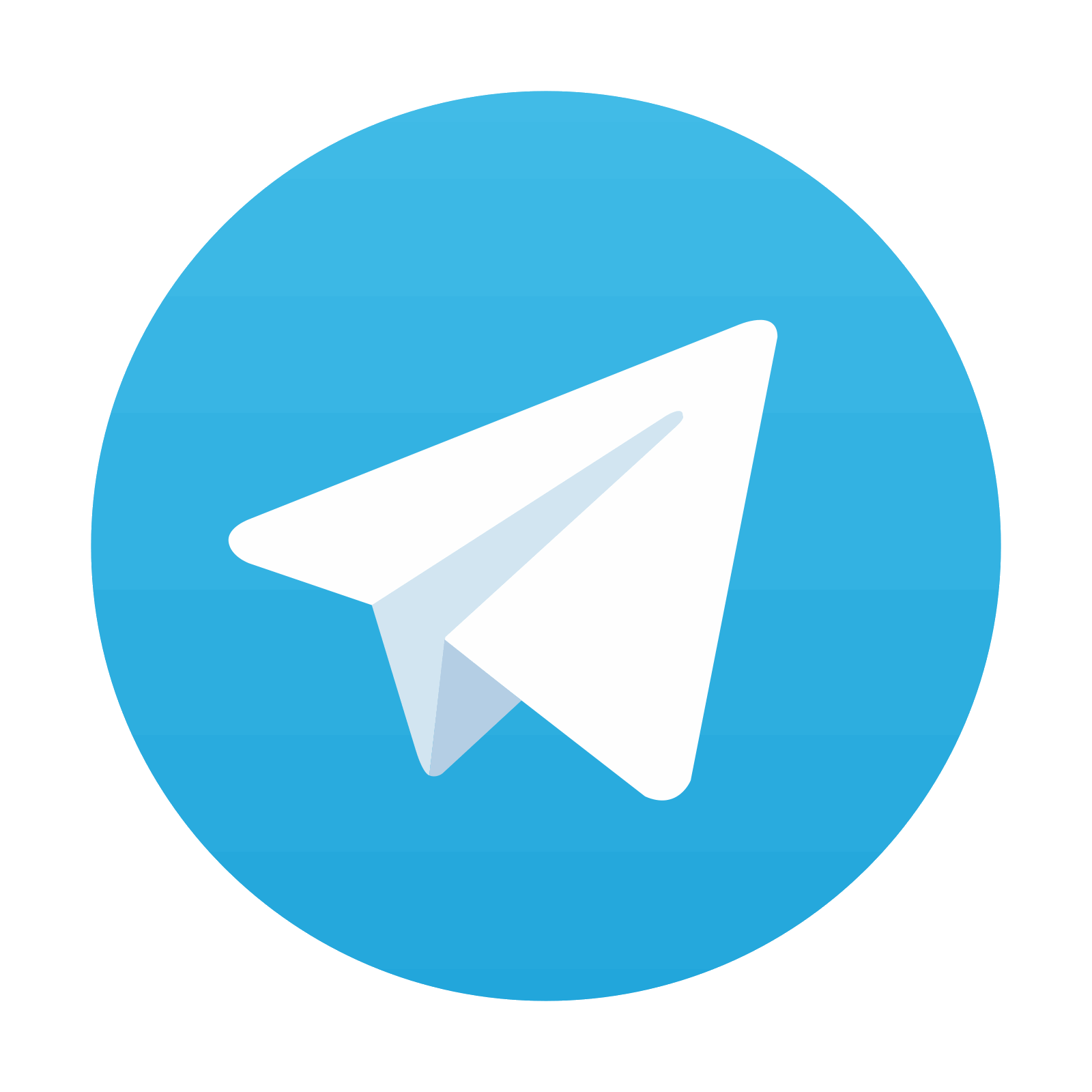
Stay updated, free dental videos. Join our Telegram channel

VIDEdental - Online dental courses
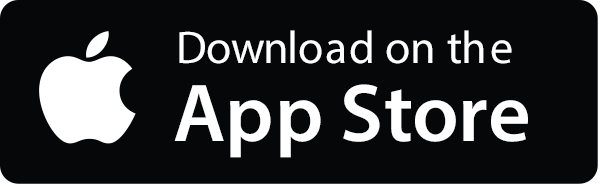
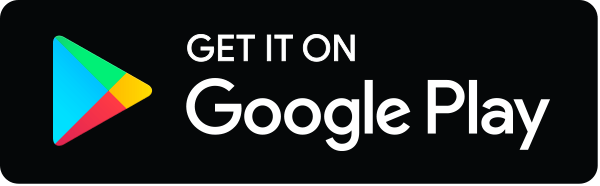