Abstract
Objectives
To demonstrate that determination of the depth of cure of resin-based composites needs to take into account the depth at which the transition between glassy and rubbery states of the resin matrix occurs.
Methods
A commercially available nano-hybrid composite (Grandio) in a thick layer was light cured from one side for 10 or 40 s. Samples were analyzed by Vickers indentation, Raman spectroscopy, atomic force microscopy, electron paramagnetic imaging and differential scanning calorimetry to measure the evolution of the following properties with depth: microhardness, degree of conversion, elastic modulus of the resin matrix, trapped free radical concentration and glass transition temperature. These measurements were compared to the composite thickness remaining after scraping off the uncured, soft composite.
Results
There was a progressive decrease in the degree of conversion and microhardness with depth as both properties still exhibited 80% of their upper surface values at 4 and 3.8 mm, respectively, for 10 s samples, and 5.6 and 4.8 mm, respectively, for 40 s samples. In contrast, there was a rapid decrease in elastic modulus at around 2.4 mm for the 10 s samples and 3.0 mm for the 40 s samples. A similar decrease was observed for concentrations of propagating radicals at 2 mm, but not for concentrations of allylic radicals, which decreased progressively. Whereas the upper composite layers presented a glass transition temperature – for 10 s, 55 °C (±4) at 1 mm, 56.3 °C (±2.3) at 2 mm; for 40 s, 62.3 °C (±0.6) at 1 mm, 62 °C (±1) at 2 mm, 62 °C (±1.7) at 3 mm – the deeper layers did not display any glass transition. The thickness remaining after scraping off the soft composite was 7.01 (±0.07 mm) for 10 s samples and 9.48 (±0.22 mm) for 40 s samples.
Significance
Appropriate methods show that the organic matrix of resin-based composite shifts from a glassy to a gel state at a certain depth. Hence, we propose a new definition for the “depth of cure” as the depth at which the resin matrix switches from a glassy to a rubbery state. Properties currently used to evaluate depth of cure (microhardness, degree of conversion or scraping methods) fail to detect this transition, which results in overestimation of the depth of cure.
1
Introduction
Photopolymerizable resin-based composites (RBC) have improved considerably since they were introduced, and are currently accepted as reliable materials for direct anterior and posterior dental restoration. Their use has now been extended to large and deep cavities , which require the build-up of RBC restorations in successive layers, first because of the limited cure depth and second to reduce the consequences of shrinkage stress . Knowledge of the maximum thickness for each layer is crucial for the clinician. Use of excessively thick layers can result in insufficient cure of the RBC at depth, leading to reduced mechanical properties and biocompatibility. The “depth of cure” (DOC) – usually referring to the thickness of a RBC that is “adequately” cured – is limited by light absorption and scatter within the material, which are influenced by numerous factors, including the amount, size and type of fillers , RBC shade , photoinitiator type and concentration , refractive index mismatch , light irradiation source and irradiation duration .
Several methods have been used to assess the DOC. A simplistic method has been described in the ISO 4049 standard and is based on measurement with a micrometer of the thickness of the RBC that remains after removal of uncured soft material with a plastic spatula . Other groups have used a penetrometer to measure the DOC based on application of a needle with a constant force to the lower side of the RBC sample . Alternative methods include measurement of the degree of conversion (DC) – by Raman or FTIR spectroscopy – or of the microhardness (MH) at regular intervals through the depth of the material. Based on these measurements, the DOC is usually described as the depth at which the MH or DC value equals the surface value multiplied by an arbitrary ratio, usually 0.8 .
Several factors suggest, however, that these methods might not be appropriate to measure the quality of cure of a RBC at depth. When considering the DC at depth, it is difficult to determine which DC corresponds to “adequate” polymerization. In fact, the DC value on its own is insufficient, as it does not provide any information on the state of network development, i.e., the degree of cross-linking. The highly crosslinked nature of dimethacrylate-based polymers is responsible for a non-linear polymerization process, marked by two macroscopic changes of state: first, gelation, which refers to the change from a viscous liquid to an elastic gel (infinite network) occurring at a relatively low DC (<10%) – the “gel point”; second, vitrification, which refers to the transformation from the rubbery material to a glassy one, accompanied by a considerable increase in elastic modulus (3–4 orders of magnitude) . The DC and the degree of cross-linking determine when the polymer network changes from a liquid to a gel, and then from a rubbery to a glassy state. These two transitions have been very well described by real-time measurement of DC in thin samples (<2 mm): as DC and crosslinking increase with irradiation time, the liquid resin transforms into a gel and then into a glassy material .
Given the logarithmic decrease in light transmittance at depth (only 25% overall transmittance at 1 mm depth, 12% at 2 mm and 7% at 3 mm) , RBC conversion and/or crosslinking decreases with depth. Hence, similar to what is observed in real-time polymerization experiments, it is conceivable that at a certain depth, the resin would first change from a glassy to a rubbery state, then from a rubbery to a liquid state. The transition between gel and liquid is relatively obvious, as a RBC with a liquid resin matrix is soft and can easily be scraped away. However, the theoretically sharp transition between rubbery and glassy polymer has to our knowledge never been described, possibly because the methods used so far have not been able to measure this event. The size of the micro-indenter, of the penetrometer tip and a fortiori of the plastic spatula are indeed disproportionate compared to what needs to be measured. In this regard, it must be remembered that the quality of polymerization concerns only the resin phase of the material, which represents 30–40 vol% of the material for the most highly filled RBCs. Hence, most of the material is composed of fillers, which are tightly packed, leaving very little space for the resin between them. As an example, the width of the indentation left by a Vickers indenter in a dental RBC is around 60 μm, which is considerably larger than the distance between two neighboring fillers that the resin occupies, i.e., less than 1 μm based on scanning electron microscopy (SEM) images . This simple comparison highlights the difficulty in assessing the properties of the resin using hardness, penetrometer and scraping measurements, and suggests that other methods, which can limit any interference of the fillers in the results, should be used.
The working hypothesis of the present study was, therefore, that determination of the DOC of a RBC needs to take into account the depth at which transition between the glassy and rubbery states of the resin matrix occurs. Specific techniques were used to highlight this transition, i.e., atomic force microscopy (AFM) to measure the elastic modulus of the resin between the fillers (E-Mod, MPa), electron paramagnetic resonance (EPR) imaging to determine the trapped radical concentration ( R , a.u.) at each depth, and differential scanning calorimetry (DSC) to determine the glass transition temperature ( T g , °C) at different depths. These results were compared to classical methods, i.e., Vickers MH, DC by Raman spectroscopy, and a “scraping” test similar to the ISO 4049 standard.
2
Materials and methods
A highly filled nano-hybrid RBC, Grandio (Voco, Cuxhaven, Germany) of shade A3 (batch #0952138, ref 1812) was chosen for all experiments. For AFM, EPR imaging, Raman spectroscopy and Vickers MH measurements, the RBC was packed into a rectangular Teflon mold of 3 mm × 3 mm aperture and 6 mm depth, covered by a polyester film and irradiated from one side. For the “scraping” tests, a similar Teflon mold was used but 10 mm deep, similar to the ISO 4049 specifications . For DSC measurements, 6 cylindrical Teflon molds (1 mm depth and 3 mm diameter) were successively filled and superposed on each other, a polyester film separating each mold from its neighbor. Regardless of the mold, the light tip of the LED light BluePhase G2 (Ivoclar-Vivadent, Schaan, Liechtenstein) was then placed against the upper surface film and polymerization was initiated using the low radiation mode (650 mW/cm 2 ) for either 10 or 40 s. In the instructions for use, the manufacturer recommends a minimum irradiation of 500 mW/cm 2 and a curing time of minimum 20 s per 2 mm layer, increased to 40 s for opaque shades. Hence, in order to insure optimal material properties, 40 s was chosen as reference in this work, as in many other published studies. 10 s irradiation time is less than the manufacturer’s recommendations but was chosen to deliberately create a difference in light curing quality at depth and to test the ability of each technique to identify the limit between optimal and sub-optimal cure.
After photopolymerization, the samples were carefully removed from the mold and stored for 24 h in the dark at room temperature (23 ± 1 °C) before analysis, to insure that the polymerization process was complete prior to analysis .
The DC was measured on the upper surface and at every millimeter on the side of the sample ( n = 3) using a Raman Spectrometer (DXR Raman Microscope, Thermo Scientific, Madison, WI, USA) . The samples were excited at 780 nm by a frequency-stabilized single mode diode laser through a microscope objective (×50) and spectra were obtained in the region 1600 cm −1 , with the following conditions: microhole, 50; irradiation time, 60 s; number of accumulations, 5; grating 400 lines/mm. The DC was then calculated as the percentage of vinyl functions converted to aliphatic functions based on the decrease in the C C bond peak at 1640 cm −1 compared to the uncured sample; the aromatic C C peak at 1610 cm −1 was used as the internal standard.
Vickers MH measurements were carried out on the upper surface and at every millimeter on the side of the sample ( n = 3) with a Durimet microhardness tester (Leitz,Wetzlar, Germany). A load of 200 g was applied for 30 s on the surface, the length of the diagonal of each indentation was measured directly using a graduated eye-lens and the Vickers hardness number (VHN) was obtained using tables, based on the following equation (average of 3 indents per sample): H = 1854.4 ( P d −2 ), where H is the Vickers hardness (kg mm −2 ), P is the load (g) and d is the diagonal length of the indent (μm).
AFM was used to evaluate the E-mod of the organic matrix between the RBC fillers. Images (50 μm × 50 μm and 10 μm × 10 μm) were acquired on the side of the sample at regular distances from the upper surface (every 250 μm) in the so-called HarmoniX™ mode using a Multimode AFM with a Nanoscope V controller (Brucker). The Harmonix™ mode allows simultaneous mapping of the topography, the phase-shift, the adhesion force, the energy dissipation at the tip-surface contact and the elastic modulus. This mapping is achieved by using special torsional probes and by reconstructing and analyzing the tip-surface force curve from the spectrum of the torsional vibration of the probe at every image point . All the images were acquired in air at ambient temperature. A Harmonix™ high modulus probe was used (spring constant 2.3 N m −1 , flexural resonance frequency 53.4 kHz and torsional resonance frequency 910 kHz). To obtain quantitative data, the probe, the photodetector sensitivity and the tip-surface transfer function were calibrated using the procedure proposed by the microscope’s manufacturer on a standard sample. This sample is a thin film of polystyrene ( E ≈ 2000 MPa) with dispersed low-density polyethylene nodules ( E ≈ 50 MPa). The images obtained (available in appendix 1 and 2 for 10 and 40 s, respectively) were treated and analyzed using home-made procedures developed under Igor Pro (Wavemetrics). The resin modulus was determined as the average value obtained on the modulus images, excluding the fillers. This was obtained by creating histograms of the moduli based on the images, and by selecting as E-mod the peak value corresponding to the resin after excluding the high modulus peak(s) corresponding to the fillers. The nano-indentation depth ranges from 1 to 2 nm and the tip radius of curvature is <10 nm. During AFM measurements, strain field might vary within inter-particulate spaces, and therefore with various filler morphologies and content. Though, as the present work was based on a single RBC, such parameter was not taken into account in our analysis. In future work comparing the position of the glassy–rubbery transition in various RBCs, AFM should remain an adequate method as well. Indeed, even if the variation of strain field might affect the modulus value of each RBC, which is of lesser interest here, it should not influence the detection of the glassy–rubbery transition.
The concentration of trapped radicals at different depths of the cured specimens ( n = 5) was measured by EPR spectral imaging. The EPR images were acquired using an EPR Elexsys E540 System (Bruker, Rheinstatten) in 2D spectral mode. The samples were positioned in the center of an X-Band EPR Super High Q cavity cylindrical resonator (ER 4122SHQE, 10 mm diameter) operating at ∼9.5 GHz. The main EPR parameters consisted of: center field, 341.890 mT; sweep width, 36.5 mT; spectral width, 20.0 mT; modulation frequency, 100 kHz; modulation amplitude 0.1 mT, microwave power, 0.5 mW. Images were reconstructed by filtered backprojection, with a Shepp-Logan filter, using the Xepr software package (Bruker). No deconvolution was applied before reconstruction. As peak width did not vary significantly with depth, measurement of the height of the EPR peaks at each depth could be used to determine the relative concentration (in arbitrary units, a.u.) of trapped radicals: height of the fifth and fourth peaks of the 9-line spectrum for allylic ( R Allyl ) and propagating radicals ( R Propag ), respectively. These peaks are influenced less by the signals of other radical types, as described previously .
The glass transition temperature ( T g ) was measured using a DSC (822e/HSS7, Mettler Toledo, Greifensee, Switzerland). Each of the six 1 mm–thick superposed layers was submitted to two successive heating procedures (run 1 and run 2) at a heating rate of 10 °C/min from −5 to 130 °C under nitrogen, separated by a cooling procedure at the same rate. As conversion reactions restart upon softening, i.e., at T g , T g was determined as the temperature at which an exothermal peak appeared during run 1, as previously described .
Finally, the thickness of 5 mm × 5 mm × 10 mm RBC samples was measured using a digital micrometer after scraping off the uncured (soft) RBC at the bottom of the sample with a plastic spatula, similar to the method described in the ISO 4049 specifications for a cylindrical mold .
2
Materials and methods
A highly filled nano-hybrid RBC, Grandio (Voco, Cuxhaven, Germany) of shade A3 (batch #0952138, ref 1812) was chosen for all experiments. For AFM, EPR imaging, Raman spectroscopy and Vickers MH measurements, the RBC was packed into a rectangular Teflon mold of 3 mm × 3 mm aperture and 6 mm depth, covered by a polyester film and irradiated from one side. For the “scraping” tests, a similar Teflon mold was used but 10 mm deep, similar to the ISO 4049 specifications . For DSC measurements, 6 cylindrical Teflon molds (1 mm depth and 3 mm diameter) were successively filled and superposed on each other, a polyester film separating each mold from its neighbor. Regardless of the mold, the light tip of the LED light BluePhase G2 (Ivoclar-Vivadent, Schaan, Liechtenstein) was then placed against the upper surface film and polymerization was initiated using the low radiation mode (650 mW/cm 2 ) for either 10 or 40 s. In the instructions for use, the manufacturer recommends a minimum irradiation of 500 mW/cm 2 and a curing time of minimum 20 s per 2 mm layer, increased to 40 s for opaque shades. Hence, in order to insure optimal material properties, 40 s was chosen as reference in this work, as in many other published studies. 10 s irradiation time is less than the manufacturer’s recommendations but was chosen to deliberately create a difference in light curing quality at depth and to test the ability of each technique to identify the limit between optimal and sub-optimal cure.
After photopolymerization, the samples were carefully removed from the mold and stored for 24 h in the dark at room temperature (23 ± 1 °C) before analysis, to insure that the polymerization process was complete prior to analysis .
The DC was measured on the upper surface and at every millimeter on the side of the sample ( n = 3) using a Raman Spectrometer (DXR Raman Microscope, Thermo Scientific, Madison, WI, USA) . The samples were excited at 780 nm by a frequency-stabilized single mode diode laser through a microscope objective (×50) and spectra were obtained in the region 1600 cm −1 , with the following conditions: microhole, 50; irradiation time, 60 s; number of accumulations, 5; grating 400 lines/mm. The DC was then calculated as the percentage of vinyl functions converted to aliphatic functions based on the decrease in the C C bond peak at 1640 cm −1 compared to the uncured sample; the aromatic C C peak at 1610 cm −1 was used as the internal standard.
Vickers MH measurements were carried out on the upper surface and at every millimeter on the side of the sample ( n = 3) with a Durimet microhardness tester (Leitz,Wetzlar, Germany). A load of 200 g was applied for 30 s on the surface, the length of the diagonal of each indentation was measured directly using a graduated eye-lens and the Vickers hardness number (VHN) was obtained using tables, based on the following equation (average of 3 indents per sample): H = 1854.4 ( P d −2 ), where H is the Vickers hardness (kg mm −2 ), P is the load (g) and d is the diagonal length of the indent (μm).
AFM was used to evaluate the E-mod of the organic matrix between the RBC fillers. Images (50 μm × 50 μm and 10 μm × 10 μm) were acquired on the side of the sample at regular distances from the upper surface (every 250 μm) in the so-called HarmoniX™ mode using a Multimode AFM with a Nanoscope V controller (Brucker). The Harmonix™ mode allows simultaneous mapping of the topography, the phase-shift, the adhesion force, the energy dissipation at the tip-surface contact and the elastic modulus. This mapping is achieved by using special torsional probes and by reconstructing and analyzing the tip-surface force curve from the spectrum of the torsional vibration of the probe at every image point . All the images were acquired in air at ambient temperature. A Harmonix™ high modulus probe was used (spring constant 2.3 N m −1 , flexural resonance frequency 53.4 kHz and torsional resonance frequency 910 kHz). To obtain quantitative data, the probe, the photodetector sensitivity and the tip-surface transfer function were calibrated using the procedure proposed by the microscope’s manufacturer on a standard sample. This sample is a thin film of polystyrene ( E ≈ 2000 MPa) with dispersed low-density polyethylene nodules ( E ≈ 50 MPa). The images obtained (available in appendix 1 and 2 for 10 and 40 s, respectively) were treated and analyzed using home-made procedures developed under Igor Pro (Wavemetrics). The resin modulus was determined as the average value obtained on the modulus images, excluding the fillers. This was obtained by creating histograms of the moduli based on the images, and by selecting as E-mod the peak value corresponding to the resin after excluding the high modulus peak(s) corresponding to the fillers. The nano-indentation depth ranges from 1 to 2 nm and the tip radius of curvature is <10 nm. During AFM measurements, strain field might vary within inter-particulate spaces, and therefore with various filler morphologies and content. Though, as the present work was based on a single RBC, such parameter was not taken into account in our analysis. In future work comparing the position of the glassy–rubbery transition in various RBCs, AFM should remain an adequate method as well. Indeed, even if the variation of strain field might affect the modulus value of each RBC, which is of lesser interest here, it should not influence the detection of the glassy–rubbery transition.
The concentration of trapped radicals at different depths of the cured specimens ( n = 5) was measured by EPR spectral imaging. The EPR images were acquired using an EPR Elexsys E540 System (Bruker, Rheinstatten) in 2D spectral mode. The samples were positioned in the center of an X-Band EPR Super High Q cavity cylindrical resonator (ER 4122SHQE, 10 mm diameter) operating at ∼9.5 GHz. The main EPR parameters consisted of: center field, 341.890 mT; sweep width, 36.5 mT; spectral width, 20.0 mT; modulation frequency, 100 kHz; modulation amplitude 0.1 mT, microwave power, 0.5 mW. Images were reconstructed by filtered backprojection, with a Shepp-Logan filter, using the Xepr software package (Bruker). No deconvolution was applied before reconstruction. As peak width did not vary significantly with depth, measurement of the height of the EPR peaks at each depth could be used to determine the relative concentration (in arbitrary units, a.u.) of trapped radicals: height of the fifth and fourth peaks of the 9-line spectrum for allylic ( R Allyl ) and propagating radicals ( R Propag ), respectively. These peaks are influenced less by the signals of other radical types, as described previously .
The glass transition temperature ( T g ) was measured using a DSC (822e/HSS7, Mettler Toledo, Greifensee, Switzerland). Each of the six 1 mm–thick superposed layers was submitted to two successive heating procedures (run 1 and run 2) at a heating rate of 10 °C/min from −5 to 130 °C under nitrogen, separated by a cooling procedure at the same rate. As conversion reactions restart upon softening, i.e., at T g , T g was determined as the temperature at which an exothermal peak appeared during run 1, as previously described .
Finally, the thickness of 5 mm × 5 mm × 10 mm RBC samples was measured using a digital micrometer after scraping off the uncured (soft) RBC at the bottom of the sample with a plastic spatula, similar to the method described in the ISO 4049 specifications for a cylindrical mold .
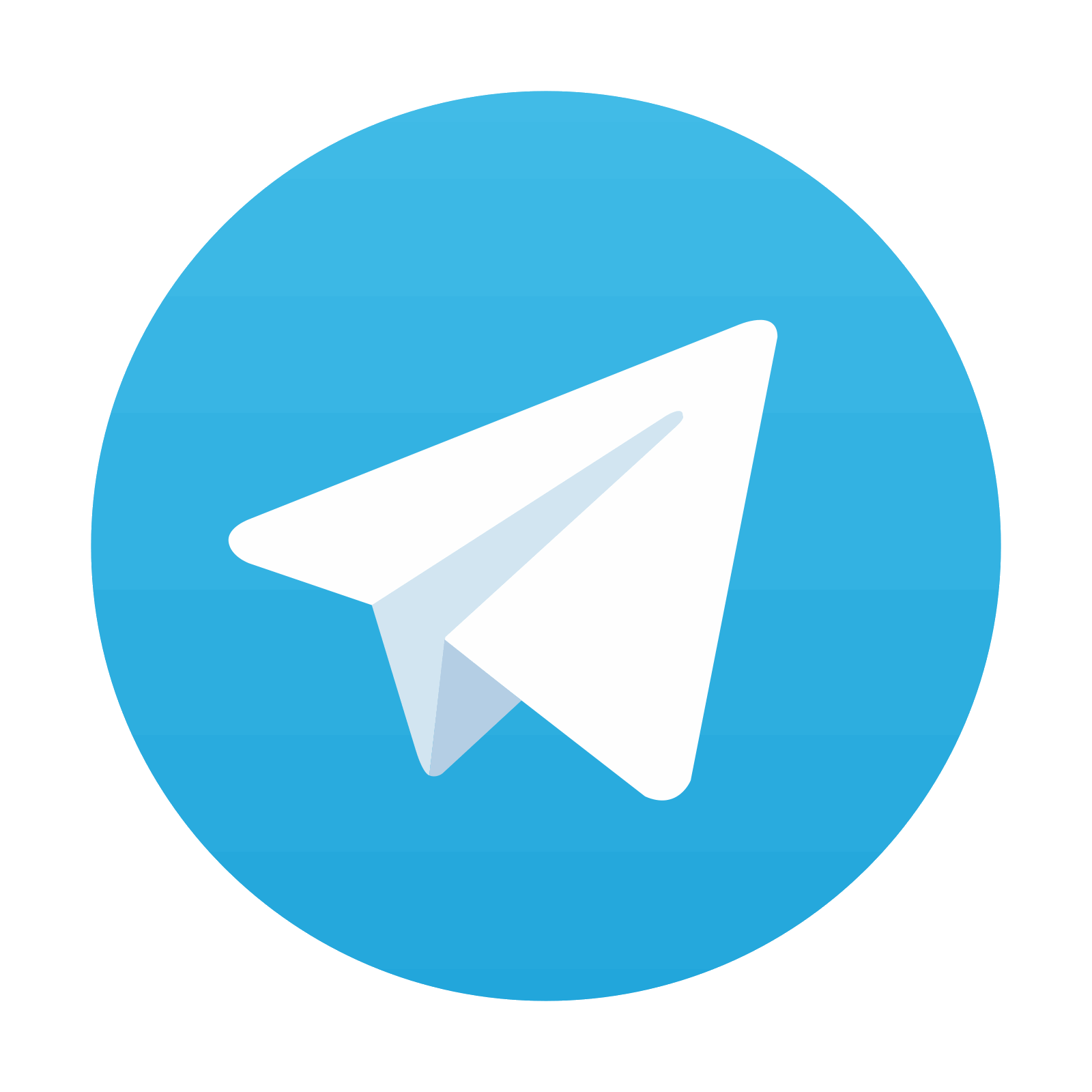
Stay updated, free dental videos. Join our Telegram channel

VIDEdental - Online dental courses
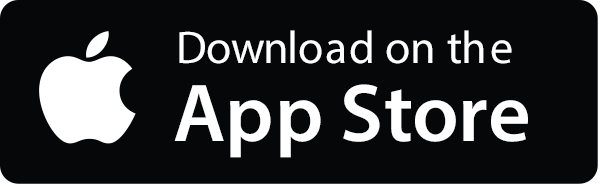
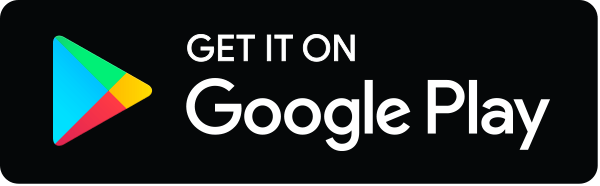