Abstract
Objective
To evaluate degree of conversion (DC), Knoop microhardness (KHN), internal marginal adaptation (IA), and polymerization shrinkage stress (PS) of one conventional and four bulk-fill composites.
Methods
Bulk-fill composites tested were Surefil SDR (SDR), Filtek Bulk-Fill (FBF), Tetric EvoCeram Bulk-Fill (TEC), and EverX Posterior (EXP). The conventional composite Herculite Classic (HER) was tested using both incremental and bulk-fill insertion techniques. Standardized Class I preparations (4-mm-depth) were made in extracted molars and restored with each product system ( N = 5). After 1-week wet storage, restorations were cross-sectioned and DC and KHN were evaluated at four depths (1, 2, 3, and 4 mm) using confocal Raman spectroscopy and KHN techniques, respectively. Epoxy resin replicas of restorations were evaluated using scanning electron microscopy for IA. PS was determined using composite bonded to acrylic rods attached to a universal testing machine ( N = 5).
Results
Within bulk-fill products, only SDR and FBF demonstrated similar DC at all depths, and KHN values did not statistically differ among depths, except for TEC. Neither placement method nor depth affected KHN or DC, except the DC of HER bulk-fill at 4 mm. Incrementally layered HER, and bulk-fills SDR and TEC demonstrated the lowest proportion of internal gaps. Highest and lowest PS values were measured for EXP and TEC, respectively.
Significance
DC with depth was not uniform among all bulk-fill materials, although no difference in KHN was found. Higher PS correlated positively with higher proportion of interfacial gaps. The incremental technique using conventional composite showed reduced gap formation.
1
Introduction
The main reasons for clinical failure of resin composite restorations are secondary caries and bulk fractures . The former are related to early gap formation and subsequent degradation of the exposed surfaces involved in restoration bonding: dentin, adhesive, and composite itself. Gap formation may be associated with volumetric changes in resin-based materials resulting from shrinkage stress (PS) during polymerization at the bonded interface . The bonding agent efficiency and attention to technical aspects of material placement also influence the longevity of restorations . The fracture strength of resin composite restorations is related to the material mechanical properties, which are dependent not only on material composition, but also upon the extent of cure (monomer conversion), and on clinical aspects such as the amount of remaining, supported tooth structure, the preparation design, and to local occlusal conditions .
Incremental filling techniques have been proposed to optimize composite polymerization and its internal marginal adaptation, using either 2-mm-thick oblique or horizontal increments . These procedures are thought to reduce the final volumetric shrinkage of the material, and thus the level of PS developed, and therefore minimize internal gap formation . Clinically, however, incremental filling techniques are complex and require significant chair-side time for restoration placement.
Recent advances in dental resin-based restorative materials have resulted in development of composites for bulk placement, to replace the need for incremental layering . Manufacturers claim that these new composites display reduced volumetric shrinkage, lower PS, and increased depth of cure, allowing for single incremental placement in up to 4-mm thick layers. Bulk-fill composites thus have the potential advantages of simplifying clinical technique and saving time. Furthermore, bulk placement prevents void incorporation and contamination between composite layers, leading to more compact fillings . Such advantages are possible because of the increased translucency of the bulk-fill composites, which allows greater light transmission . In addition, the formulation of these materials allows for modulation of the polymerization reaction by use of special, stress-relieving monomers, the use of more reactive photoinitiators, and the incorporation of different types of fillers, such as pre-polymer particles and fiberglass rod segments.
Several studies demonstrate favorable outcomes for bulk-fill composites, with respect depth of cure. These studies used spectroscopic methods, such as Fourier transform infrared and Raman spectroscopy , to determine the degree of conversion (DC). Microhardness measurements are used as an indirect method for evaluating DC . However, this method has been questioned, because it overestimates the depth of cure . As a result of differences in experimental methods and in data analysis, evaluation of polymerization shrinkage of bulk-fill composite remains controversial . Furthermore, few studies have focused on the PS of bulk-fill composites and its potential influence on clinically related outcomes . One 3-year long clinical evaluation compared the performance of a bulk-fill composite to a 2-mm-thick, incrementally placed resin composite. The results indicated that the bulk-fill material performed just as effectively as the incrementally placed product . For a clinician to confidently change from using a traditional, incremental filling technique to the bulk-filling method depends on providing further, convincing clinical trials and laboratory studies that compare characteristics of the polymerization reaction at restoration depths as well as contrast physical properties that simulate the clinical scenario.
The purpose of this study was to evaluate the effect of composite depth on degree of monomer conversion (DC) and microhardness (Knoop (KHN)), as well as the measurement of proportion of discontinuous bonded interfacial locations (internal marginal adaptation (IA)) when restoring controlled-size preparations in extracted teeth, and its correlation with polymerization stress (PS) among the different products. As control, a conventional, incremental composite was used in both its recommended manner (positive control) as well as in bulk placement (negative control). The following null hypotheses were tested: (1) Within a given composite, there would be no significant difference in either DC or KHN with increasing composite depth; and (2) there would be no significant difference in either interfacial gap proportion or maximum polymerization stress among the bulk-fill products, and the conventional, layered composite control material.
2
Materials and methods
Five commercial resin-based composites were investigated: one conventional product (Herculite Classic—HER) applied both incrementally (positive control) and in bulk (negative control), two high-viscosity, bulk-fill composites (Tetric EvoCeram Bulk Fill (TEC) and EverX Posterior (EXP)), and two flowable, bulk-fill composites (Surefil SDR Flow (SDR) and Filtek Bulk Fill (FBF)). For each material, the respective adhesive system was used during the restorative procedures. Product specifications are presented in Table 1 .
Classification | Abbreviation used in text | Brand name (lot number) | Adhesive system (lot number) | Manufacturer | Matrix composition | Filler type | Filler loading (volume %) | Shade |
---|---|---|---|---|---|---|---|---|
Conventional incrementally layered composite | HER | Herculite Classic (400936) | Gel Etchant (31297) OptiBond FL (31297) |
Kerr Co, Orange, CA, USA | Bis-GMA, TEGDMA | Borosilicate-aluminum glass | 59 | A2 |
Bulk-fill: flowable | SDR | Surefil SDR flow (08153) | Etching Dental Gel (671857E) XP Bond (793475F) |
Dentsply Caulk, Mildford, DE, USA | Modified UDMA, TEGDMA, EBPDMA | Barium-aluminofluoro-borosilicate glass, strontium-aluminofluoro-borosilicate glass | 44 | Universal |
FBF | Filtek Bulk Fill (402919) | Scotchbond Universal (482988) | 3M ESPE, St. Paul, MN, USA | Bis-GMA, Bis-EMA, UDMA, TEGDMA, Procrylat resins | Zirconia/silica, ytterbium trifluoride | 42.5 | A2 | |
Bulk-Fill: high-viscosity | TEC | Tetric EvoCeram Bulk Fill (R04686) | Total Etch Gel (R39845) Tetric N-Bond (R27602) |
Ivoclar Vivadent AG, Schaan, Liechtenstein | Bis-GMA, UDMA | Barium glass, ytterbium trifluoride, oxides and prepolymers | 60 | IVA |
EXP | EverX Posterior (1401152) | G-aenial Bond (1205231) | GC Corporation, Tokyo, Japan | Bis-GMA, TEGDMA, PMMA | Hybrid filler, fractions and E-glass fibers | 57 | Universal |
A polywave light emitting diode curing unit (VALO, Ultradent Products Inc., South Jordan, UT, USA) was used for all of light-curing procedures. The irradiance of the unit between 350 and 550 nm was 995 ± 2 mW/cm2, as measured using a 6″ integrating sphere (CTSM-LSM-60-SF, Labsphere Inc., N. Sutton, NH USA) and laboratory-grade spectral radiometer (USB 2000, Ocean Optics, Dunedin, FL USA). Prior to measurement, the system was calibrated using a NIST-traceable light source. The composites were light-cured following manufacturer recommendations, with the emitting end of the light source as close to the upper composite surface as possible, without actually touching it: 20 s for the bulk-fill composites and 40 s for the regular composite.
2.1
Cavity preparation and restoration placement
Thirty freshly extracted, caries-free, human third molars were collected and stored in an aqueous, 0.2% thymol solution at 4 °C, to minimize bacterial contamination, for up to 1 month after extraction. The teeth were obtained and used in accordance with a previously approved protocol (No. 015/2014) from the Research Ethics Committee of Piracicaba Dental School, State University of Campinas, Piracicaba, Brazil.
Tooth cusps were abraded using silicon carbide abrasive paper (grit No. 320) in order to obtain a flat enamel occlusal surface. Standardized Class I, box-shaped preparations were manually made, providing a mesio-distal width 4.0 mm, a bucco-lingual width 3.0 mm, and a depth of 4.0 mm, having a C-factor of 5.7 and an approximate volume of 48 mm3. A periodontal probe was used to verify preparation dimensions during fabrication. Preparations were made using regular and fine diamond burs (nos. 3145 and 3145FF, respectively, KG Sorensen, Cotia, SP, Brazil) in a high-speed handpiece (Kavo, Joinville, SC, Brazil) with water-cooling. All preparation cavosurface margins were surrounded by enamel. The prepared teeth were divided randomly into 6 groups ( N = 5), according to the restorative system and filling technique.
The specific adhesive systems corresponding to each commercial composite product were applied following manufacturer instructions. The restorative systems, (composite/adhesive) consisting of Herculite Classic/OptiBond, Surefil SDR Flow/XP Bond, and Tetric EvoCeram Bulk Fill/Tetric N-Bond, were applied using the total-etch bonding technique, while Filtek Bulk Fill/Scotchbond Universal and EverX Posterior/G-aenial Bond used self-etching adhesive systems. The conventional composite, Herculite Classic, was tested using two filling techniques: incremental and bulk-fill. In the incremental technique, an oblique incremental technique was used. The composite was applied in four, wedge-shaped increments (each approximately 2.0 mm thick), and each layer was individually light-cured for 40 s. When placed using the bulk-fill mode, the 4-mm increment of HER was light-cured for 40 s only once. For all bulk-fill products (SDR, FBF, TEC, and EXP), the preparation was filled in one single increment and light-cured for 20 s.
After storage for one week at 37 °C in distilled water, the restored teeth were cross-sectioned through their centers in a bucco-lingual direction, using a diamond blade (Isomet Diamond Wafering Blades, no. 11-4244, Buehler Ltd., Lake Buff, IL, USA) with water-cooling. Both halves were polished using a sequence of silicon carbide abrasive papers (grits no. 1000, 1200, and 2000, Norton Abrasivos, Vinhedo, SP, Brazil) and felt disks containing 3, 2, 1, and 0.5 μm diamond pastes (Buehler Ltd.). Specimens were sonicated (Thornton USC 1400, Unique Group, Indaiatuba, SP, Brazil) for 10 min in order to remove the polishing debris.
The restored, sectioned, and polished teeth were divided for evaluation using different analytical techniques: one half side was directly subjected to confocal Raman spectroscopic analysis and Knoop microhardness testing (non-destructive analyses), and the other half was replicated in epoxy and used to evaluate the internal marginal adaptation of the restorations via scanning electron microscopy (SEM).
2.2
Confocal Raman spectroscopy
The sectioned composite resin restoration surfaces were analysed using confocal Raman spectroscopy in order to determine DC at different depths. Three measurements were performed each at 1, 2, 3, and 4 mm depths. Raman spectra were collected using a spectrometer (Skin Analyzer—model 3510, River Diagnosis BV, Rotterdam, Netherlands) with a diode laser at a wavelength of 785 nm. The software (River Icon, River Diagnosis BV, Rotterdam, Netherlands) generated three individual spectra at each measurement location, each using 10-s exposure. Laser penetration depth into the composite surface was controlled at 150 μm. To obtain spectra of the uncured materials, the composite resins were each placed in an aluminium rod sample holder and spectra were collected using the same procedure described above.
Raman data were analyzed between 1570 and 1660 cm−1. The spectra were processed (minimum–maximum normalization (baseline-correction), background correction, and wavelength range selection) using software (OPUS v. 4.2, Bruker Optik GmbH, Ettlingen, Germany). The Raman vibrational stretching modes at 1610 and 1640 cm−1 were fitted with Lorentzian shapes in order to obtain the absorption peak heights using a different software package (Origin, Microcal Software Inc., Northampton, MA, USA). The DC values were calculated from changes in the ratios of peak heights of the aliphatic C = C bond (1640 cm−1) and the aromatic C C bond (1610 cm−1) in the cured and uncured specimens, using the following equation :
DC % = 100 × 1 − R cured R uncured
where R is the absorption peak height ratio at 1640 cm−1/peak height at 1610 cm−1.
The monomer conversion values of the three individual spectra obtained at each location were averaged into a single value and were used to represent the DC of that specific specimen at the identified depth location.
2.2.1
Knoop microhardness
The same specimen locations analyzed using confocal Raman spectroscopy were also used to determine KHN values. A microhardness tester (HMV 2000, Shimadzu, Tokyo, Japan) with a Knoop diamond indenter was used to apply a static load of 100 g (0.98 N) for 10 s to each composite surface. For each specimen, the averages of three indentations at each depth were used in the statistical analysis.
2.2.2
Internal marginal adaptation
Impressions of the polished, half-restored surfaces were taken using a polyvinyl siloxane material with light and heavy consistencies materials (Express XT, 3M ESPE, St. Paul, MN, USA) and were poured with an epoxy resin (EpoxiCure, Buehler Ltd.). The polymeric replicas were sputter-coated with gold to a thickness of approximately 50 Å in a vacuum evaporator (SCD 050, Bal-Tec AG, Balzers, Liechtenstein) in order to analyze the presence of internal interfacial gaps in a scanning electron microscope (SEM) (JEOL, JSM-5600LV, Tokyo, Japan).
For each specimen, approximately 40 images, each at a 200× magnification, were required to observe the entire length of the bonded internal interface. Software (ImageJ, National Institute of Health, Bethesda, USA) was used to determine the length of individual debonded segments along the entire internal perimeter of the restoration. The SEM image scale bar was used for distance calibration. Individual lengths of debonded segments were obtained in millimetres, were summed, and then converted to percentage of the total length of the interface.
2.3
Polymerization stress
Polymethyl methacrylate rods (4 mm OD) were sectioned into lengths of 13 and 28 mm. One surface of the 13-mm long rods was polished using a series of silicon carbide abrasive papers (grits no. 600, 800, 1000, and 1200, Norton Abrasivos, Vinhedo, SP, Brazil) and felt disks with 1 μm diamond paste (Buehler Ltd.) in order to optimize the transmission of light through the rod end into the specimen during photoactivation. The opposite surfaces of the 13 mm rods and both the surfaces of the 28 mm rods were sandblasted using 100 μm aluminium oxide particles (BioArt, São Carlos, SP, Brazil). Methylmethacrylate monomer (JET Acrílico Auto Polimerizante, Artigos Odontológicos Clássico, São Paulo, SP, Brazil) was then applied on the sandblasted surfaces followed immediately by two layers of unfilled resin (Scotchbond Multipurpose Plus, bottle 3, 3M ESPE, St. Paul, MN, USA) and light-cured for 10 s.
The rods were subsequently attached to a universal testing machine (model 5565, Instron Corp, Canton, MA, USA). The 13 mm rods were attached to the lower clamp and the 28 mm rods to the upper clamp. Composite was placed on the etched and bonded 13-mm rod end, and the activator moved the upper rod downward, leaving a controlled distance between at the rod ends of 0.8 mm. Excess composite was removed, and the periphery of remaining composite was smoothed to match the outline of the acrylic rod sides. The resulting composite volume was 6.8 mm3, having a C-Factor of 2.5. An extensometer (model 2630–101, Instron Corp) was attached to the rods, to monitor specimen height and provide feedback to the testing machine in order to move the actuator such that the rod separation distance (specimen height) was maintained during polymerization. The tip of the light-curing unit was positioned in contact with the polished surface of the 13 mm rod and turned on. Values registered by the load cell corresponded to the force necessary to counteract the polymerization shrinkage force and to thus maintain the initial specimen height. Force development was monitored for 10 min from the beginning of light-activation, and the maximum nominal stress (MPa) was calculated by dividing the maximum force value recorded by the cross-sectional area of the rods. Five specimens were tested for each material.
2.4
Statistical analyses
Because neither DC nor KHN values can be validly compared among products, these parameters were analyzed separately using the split-plot, one-way ANOVA: factor: depth, at 4 levels. However, because the same commercial product (HER) was used for both incremental and bulk-fill addition, a 2-way ANOVA was performed only for this material to examine the influence of both placement technique (2 levels) and depth (4 levels) for both DC and KHN values. The IA and PS data were evaluated using one-way ANOVA tests (factor: material (5 levels). Where appropriate, Tukey post-hoc tests were applied in order to detect pair-wise mean differences among the groups ( α = 0.05).
Pearson’s test was also used to detect the presence of statistically significant correlations between the DC and KHN data. The correlation between proportion of internal gaps and polymerization stress values was evaluated using linear regression. For all statistical testing, a pre-set, global significance level of 5% was used.
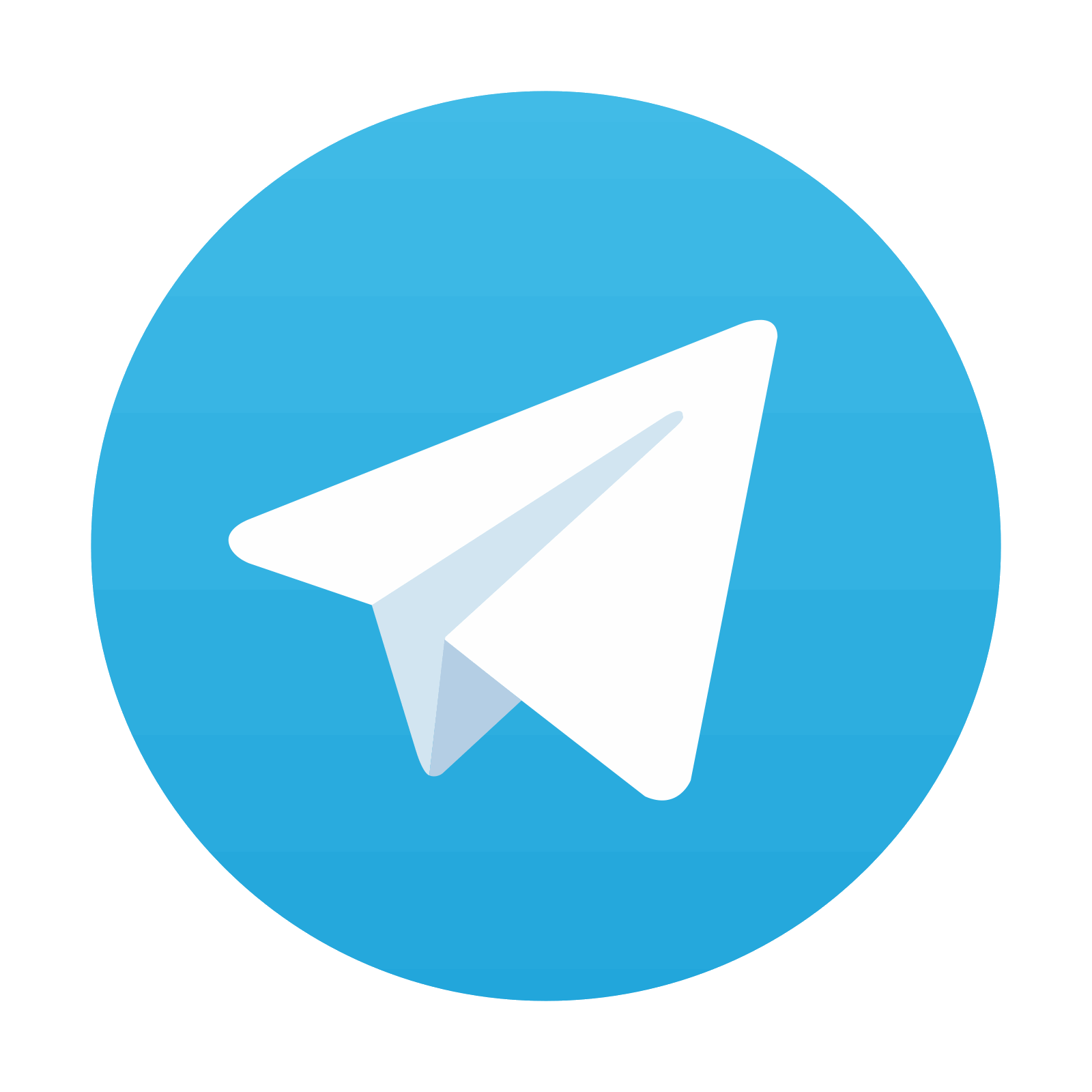
Stay updated, free dental videos. Join our Telegram channel

VIDEdental - Online dental courses
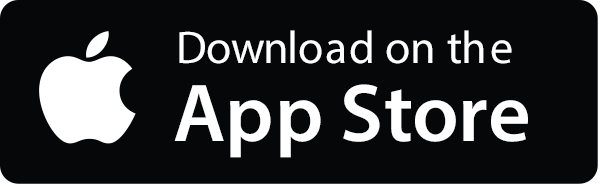
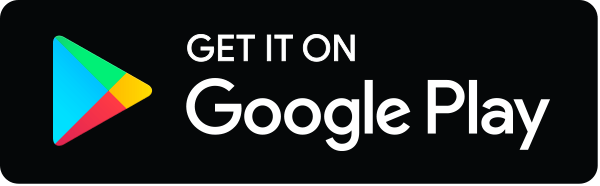