Abstract
Objectives
Two types of ceramic coatings on commercially pure titanium for dental implant applications with different Ca/P ratios in the range from 1.5 to 4.0, and two different thicknesses (∼5 and ∼15 μm) were examined with the aim of underpinning the effect of coating composition, thickness and microstructure on the corrosion behavior and hydroxyapatite forming ability in SBF.
Methods
Bioactive coatings were formed on Ti by plasma electrolytic oxidation (PEO). The composition, structure, and morphology of the materials were characterized before and after the immersion in simulated body fluid solution (SBF) at 37 °C for up to 4 weeks. All the materials were screened with respect to metal ion release into SBF.
Results
Only thick PEO coating with overstoichiometric Ca/P ratio of 4.0 exhibited capacity to induce the precipitation of hydroxyapatite over the short period of 1 week. Long term Ti 4+ ion release from all PEO-coated materials was 2–3 times lower than from the uncoated Ti. Metal ion release is attributed mostly to chemical dissolution of the coating at initial stages of immersion.
Significance
The long term stability was greater for thin PEO coating with overstoichiometric Ca/P ratio of 2.0, which exhibited ∼95 ng cm −2 of Ti 4+ ions release over 4 weeks. Thin PEO coatings present economically more viable option.
1
Introduction
Titanium and its alloys are well known as artificial biomaterials used for manufacturing of dental and orthopedic implants due to their high corrosion resistance and good mechanical stability . Their biocompatibility is related to the chemical stability and structural integrity of the surface oxide layer (TiO 2 ) . However, the thin native oxide layer does not form a strong bond with human bones since it is bio-inert .To overcome this drawback, the structure, composition, and chemistry of the titanium surface needs to be modified .
Plasma electrolytic oxidation (PEO), also known as micro-arc oxidation (MAO), is a relative newcomer in the biomedical field, a convenient technique for forming porous ceramic-like bioactive coatings on surfaces of titanium . The porous oxide layers containing a mixture of anatase and rutile as well as bioactive elements, such as Ca, P, Si, Mg and Ag originating from the electrolyte, accelerate calcium phosphate formation on their surface in simulated body fluid (SBF) and promote the formation of hydroxyapatite (HA) . A further advantage of PEO coatings is their good adhesion, since coating formation involves conversion (oxidation) of the substrate . In addition, both in vitro and in vivo evaluations have revealed that PEO treatment considerably improves the bioactivity of titanium and results in accelerated osseointegration of an implant. The latter is being attributed to specific topography of PEO coatings as well as to the presence of Ca and P in their composition. Since one of the characteristics of the bioactivity of a metallic implant for bone reconstruction is its apatite-forming ability in simulated body fluid, latest research on bioactive PEO coatings focuses in the generation of HA or compounds which act as precursors of HA using one-step approach, where Ca–P phases are generated during PEO, or involving post-treatments .
One of the most important characteristics of an implant is its service life, which must be extended as long as possible. This will largely depend on the corrosion resistance of the metal implant material and the release of metal ions: these are the critical factors that can adversely affect the mechanical integrity and biocompatibility . It has been reported that the stability of the titanium surface oxide layer may be affected in physiological environments in vivo , increasing the metal ion release. This has been a source of concern due to potentially harmful effect of Ti 4+ ions in vivo , because they have been shown to affect both proliferation and synthesis of extracellular matrix in vitro . Therefore, the electrochemical behavior of titanium and its alloys in biological media is of increasing interest.
Several types of physiological fluids such as SBFs, artificial saliva and phosphate buffer solutions are used in electrochemical studies performed at body temperature ( Table 1 ). In these biological media, PEO coatings have shown higher corrosion resistance than unmodified titanium alloy substrates . The composition and, in particularly, the thickness of bioactive PEO coatings may vary greatly in different studies. Even in case of today’s only commercial PEO-coated dental implant TiUnite® (Nobel Biocare), which contains about 5–8% of phosphorus, the thickness, reportedly, varies from ∼2 to ∼9 μm . Due to the mechanism of PEO coatings formation, their composition will depend on the treatment time, and therefore, on the coating thickness. The fabrication of thicker coatings requires greater energy consumption, and consequently, is more costly.
Material | Physiological solution | Reference |
---|---|---|
Ti6 A4lV | SBF | |
Ti–23Nb–0.7Ta–2Zr–O | Ringer’s solution | |
Ti6Al4V and Ti6Al6Nb | Hank’s balanced salt solution | |
Ti–6Al–4V and Ti–6Al–7Nb | Hank’s naturally aerated solution | |
Ti–Mo | Fluoridated physiological serum | |
Ti CP, Ti6 A4lV and Ti-15Mo | Ringer’s solution | |
Ti–6Al–4V | SBF | |
Ti | Ringer’s solution | |
Ti | Artificial saliva | |
Ti | Artificial saliva | |
Ti | Fusayama and Meyer’s artificial saliva | |
Ti–0.5Si–0.65C | Phosphate buffered saline | |
Sinteredporous Ti–10Mo | SBF | |
Ti–6Al–4V | Ringer’s solution |
In this study two types of PEO coatings on commercially pure titanium with different Ca/P ratios and two different thicknesses each, are being examined with the aim of underpinning the effect of coating composition, thickness and microstructure on the corrosion behavior and hydroxyapatite forming ability in SBF.
2
Experimental methods
2.1
Materials
Specimens of dimensions 30 × 20 × 0.5 mm were cut from commercially pure (c.p.) Grade I titanium foil (0.2 Fe, 0.18 O, 0.03 N, 0.015 H, and 0.18 C (max wt%)), degreased in isopropanol and rinsed in distilled water. Following subsequent pickling for ∼20 s in a mixture containing 12 mL HF (40 wt%), 40 mL HNO 3 (70 wt%) and 48 mL H 2 O at room temperature, the specimens were rinsed in distilled water, dried and masked with Lacquer 45 resin (McDermid plc.) to isolate a working area of 3 cm 2 .
2.2
Surface treatment
PEO treatment was carried out for 90 and 600 s using a 2 kW regulated AC power supply (EAC-S2000, ET Systems electronic). A square waveform voltage signal was applied with a positive-to-negative pulse ratio of 490 V/60 V at 50 Hz frequency and initial ramp of 60 s to achieve the voltage amplitude. The root mean square (rms) current density limit was set at 400 mA cm −2 , while the voltage peak-to-peak value was maintained constant. The rms voltage and current responses were acquired electronically, with a sampling time of 0.1 s, employing a Keithley KUSB-3116 data acquisition card (16 bit, 500 kS s −1 ) and Labview program (National Instruments). Instantaneous voltage and current values were monitored and recorded using a 2-channel Tektronix TDS 2012B oscilloscope at 100 MHz sampling rate.
PEO1 coating was generated in the electrolyte based on calcium acetate and sodium hexametaphosphate with ratio of Ca/P = 2; PEO2 coating was formed in calcium acetate and sodium dihydrogen phosphate solution with Ca/P = 5. Both electrolytes were suspensions with pH 7.0. The treatment was performed in a 1 L double-walled cell with re-circulating cooling system that maintained the temperature of the electrolyte at 20 °C. The cathode was made of AISI 316 stainless steel and had dimensions of 7.5 × 15 cm. After PEO, the specimens were rinsed in distilled water and dried in warm air.
2.3
Surface characterization
Coating thicknesses were measured by the eddy current method, using a Fischer ISOSCOPE FMP10 portable instrument, taking the average of ten measurements with a standard deviation of ∼0.5 μm and later confirmed with cross-sectional scanning electron microscopy (SEM). Plan views and cross-sections of coatings were examined by SEM, using a JEOL JSM-6400 microscope equipped with Oxford Link energy dispersive X-ray (EDX) microanalysis hardware. EDX surface area analysis results are cited as an average of three measurements performed at different locations. Cross-sections were prepared by grinding through successive grades of silicon carbide paper, with final polishing to a 1 μm diamond finish. Phase composition was examined by X-ray diffraction (XRD), using a Philips X’Pert diffractometer (Cu K α = 1.54056 Å) at a scanning speed of 0.01° per second for a scan range of 2 θ from 10° to 80°. Surface hardness was measured on polished cross-sections applying a load of 0.05 kg for 15 s using an AKASHI MVK-E3 Vickers microhardness machine. The cited values are the average of ten measurements. Roughness parameter R a (arithmetic average of the absolute profile deviations within the scanning path) was obtained using a Surtronic 25 roughness tester (Taylor Hobson) and TalyProfile software applying a Gaussian filter of 0.4 mm. The presented values are the average of 5 measurements performed over a distance of 4 mm. The pore population density and pore size of the coatings have been estimated using UTHSCSA Image Tool software.
2.4
Immersion tests
Coated and untreated substrates of c.p. Ti were exposed to a simulated body fluid solution (SBF, Table 2 ) as in with a pH 7.4 for times up to 4 weeks. Each specimen was immersed in 25 mL of the solution, placed in a tightly-sealed container, and thermostated at 37 °C. Two specimens for each type of material were used for reproducibility.
Reagents | Purity (%) | m-SBF a |
---|---|---|
NaCl | >99.5 | 5.403 g |
NaHCO 3 | >99.5 | 0.504 g |
Na 2 CO 3 | >99.5 | 0.426 g |
KCl | >99.5 | 0.225 g |
K 2 HPO 4 ·3H 2 O | >99.0 | 0.230 g |
MgCl 2 ·6H 2 O | >98.0 | 0.311 g |
0.2 M–NaOH | – | 100 mL b |
HEPES c | >99.9 | 17.892 g b |
CaCl 2 | >95.0 | 0.293 g |
Na 2 SO 4 | >99.0 | 0.072 g |
1.0 M–NaOH | – | 15 mL |
c HEPES = 2-(4-(2-hydroxyethyl)-1-piperaziny) ethanesulfonic acid.
b HEPES previously was dissolved in 100 mL of 0.2 M-NaOH aqueous solution.
a Buffered at pH 7.4 at 36.5 °C with HEPES and 1.0 M-NaOH aqueous solution.
2.5
Electrochemical tests
DC and AC electrochemical tests were carried out using an AUTOLAB PGSTAT30 (Eco Chemie) potentiostat in naturally aerated SBF solution at 37 °C. All measurements were reproduced at least twice. Potentials were measured with respect to a Ag/AgCl reference electrode. Solution concentration inside the reference electrode compartment was 3 M KCl, providing a potential of 0.210 V with respect to the standard hydrogen electrode. A platinum foil (∼1 cm 2 ) was used as the auxiliary electrode. The specimens were polarized at a rate of 0.3 mV s −1 from −250 to +3500 mV relative to the OCP. Electrochemical impedance spectroscopy (EIS) or, AC measurements, were carried out applying a sinusoidal perturbation of 10 mV amplitude and a frequency sweep from 10 5 to 10 −2 Hz. The frequency response was analyzed using ZView software, the goodness of fit of the simulated spectra corresponded to chi-squared (square of the standard deviation between the original data and the calculated spectrum) values <0.01. The errors for the individual parameters of the equivalent electrical circuits (such as CPE and R ) were <5%.
2.6
Ion release analysis
The immersion test solutions were acidified with 200 μL of nitric acid (65 wt%) to dissolve precipitated titanium hydroxide. After filtering through a nylon filter with 22 μm pore size and 1:6 dilution in Millipore water, the samples were analyzed by inductively coupled plasma mass spectroscopy (ICP–MS) using an Agilent 7700 ICP–MS instrument equipped with concentric quartz nebulizer and Peltier-cooled nebulizing camera. The instrument was operated in standard mode, without using the reaction cell.
Argon was used as plasma maintaining carrier gas. ICP–MS operating conditions were as follows: forward power 1550 W, argon flow 0.99 L min −1 , nebulizer pump 0.3 rps.
Three titanium isotopes were measured simultaneously ( 47 Ti, 48 Ti and 49 Ti). Calibrations based on 47 Ti were made using both deionized water and 1:6 diluted SBF-blank solution in the range from 0 to 50 μg L −1 . The calibration curve was corrected with respect to a background level of Ti detected in blank SBF (22.7 ppb). The other two isotopes reflected to a greater ( 48 Ti) or lesser ( 49 Ti) extent the effects of the SBF-matrix. The results are cited as an average of two measurements with the errors representing the deviation of the maximum and minimum from the mean.
2
Experimental methods
2.1
Materials
Specimens of dimensions 30 × 20 × 0.5 mm were cut from commercially pure (c.p.) Grade I titanium foil (0.2 Fe, 0.18 O, 0.03 N, 0.015 H, and 0.18 C (max wt%)), degreased in isopropanol and rinsed in distilled water. Following subsequent pickling for ∼20 s in a mixture containing 12 mL HF (40 wt%), 40 mL HNO 3 (70 wt%) and 48 mL H 2 O at room temperature, the specimens were rinsed in distilled water, dried and masked with Lacquer 45 resin (McDermid plc.) to isolate a working area of 3 cm 2 .
2.2
Surface treatment
PEO treatment was carried out for 90 and 600 s using a 2 kW regulated AC power supply (EAC-S2000, ET Systems electronic). A square waveform voltage signal was applied with a positive-to-negative pulse ratio of 490 V/60 V at 50 Hz frequency and initial ramp of 60 s to achieve the voltage amplitude. The root mean square (rms) current density limit was set at 400 mA cm −2 , while the voltage peak-to-peak value was maintained constant. The rms voltage and current responses were acquired electronically, with a sampling time of 0.1 s, employing a Keithley KUSB-3116 data acquisition card (16 bit, 500 kS s −1 ) and Labview program (National Instruments). Instantaneous voltage and current values were monitored and recorded using a 2-channel Tektronix TDS 2012B oscilloscope at 100 MHz sampling rate.
PEO1 coating was generated in the electrolyte based on calcium acetate and sodium hexametaphosphate with ratio of Ca/P = 2; PEO2 coating was formed in calcium acetate and sodium dihydrogen phosphate solution with Ca/P = 5. Both electrolytes were suspensions with pH 7.0. The treatment was performed in a 1 L double-walled cell with re-circulating cooling system that maintained the temperature of the electrolyte at 20 °C. The cathode was made of AISI 316 stainless steel and had dimensions of 7.5 × 15 cm. After PEO, the specimens were rinsed in distilled water and dried in warm air.
2.3
Surface characterization
Coating thicknesses were measured by the eddy current method, using a Fischer ISOSCOPE FMP10 portable instrument, taking the average of ten measurements with a standard deviation of ∼0.5 μm and later confirmed with cross-sectional scanning electron microscopy (SEM). Plan views and cross-sections of coatings were examined by SEM, using a JEOL JSM-6400 microscope equipped with Oxford Link energy dispersive X-ray (EDX) microanalysis hardware. EDX surface area analysis results are cited as an average of three measurements performed at different locations. Cross-sections were prepared by grinding through successive grades of silicon carbide paper, with final polishing to a 1 μm diamond finish. Phase composition was examined by X-ray diffraction (XRD), using a Philips X’Pert diffractometer (Cu K α = 1.54056 Å) at a scanning speed of 0.01° per second for a scan range of 2 θ from 10° to 80°. Surface hardness was measured on polished cross-sections applying a load of 0.05 kg for 15 s using an AKASHI MVK-E3 Vickers microhardness machine. The cited values are the average of ten measurements. Roughness parameter R a (arithmetic average of the absolute profile deviations within the scanning path) was obtained using a Surtronic 25 roughness tester (Taylor Hobson) and TalyProfile software applying a Gaussian filter of 0.4 mm. The presented values are the average of 5 measurements performed over a distance of 4 mm. The pore population density and pore size of the coatings have been estimated using UTHSCSA Image Tool software.
2.4
Immersion tests
Coated and untreated substrates of c.p. Ti were exposed to a simulated body fluid solution (SBF, Table 2 ) as in with a pH 7.4 for times up to 4 weeks. Each specimen was immersed in 25 mL of the solution, placed in a tightly-sealed container, and thermostated at 37 °C. Two specimens for each type of material were used for reproducibility.
Reagents | Purity (%) | m-SBF a |
---|---|---|
NaCl | >99.5 | 5.403 g |
NaHCO 3 | >99.5 | 0.504 g |
Na 2 CO 3 | >99.5 | 0.426 g |
KCl | >99.5 | 0.225 g |
K 2 HPO 4 ·3H 2 O | >99.0 | 0.230 g |
MgCl 2 ·6H 2 O | >98.0 | 0.311 g |
0.2 M–NaOH | – | 100 mL b |
HEPES c | >99.9 | 17.892 g b |
CaCl 2 | >95.0 | 0.293 g |
Na 2 SO 4 | >99.0 | 0.072 g |
1.0 M–NaOH | – | 15 mL |
c HEPES = 2-(4-(2-hydroxyethyl)-1-piperaziny) ethanesulfonic acid.
b HEPES previously was dissolved in 100 mL of 0.2 M-NaOH aqueous solution.
a Buffered at pH 7.4 at 36.5 °C with HEPES and 1.0 M-NaOH aqueous solution.
2.5
Electrochemical tests
DC and AC electrochemical tests were carried out using an AUTOLAB PGSTAT30 (Eco Chemie) potentiostat in naturally aerated SBF solution at 37 °C. All measurements were reproduced at least twice. Potentials were measured with respect to a Ag/AgCl reference electrode. Solution concentration inside the reference electrode compartment was 3 M KCl, providing a potential of 0.210 V with respect to the standard hydrogen electrode. A platinum foil (∼1 cm 2 ) was used as the auxiliary electrode. The specimens were polarized at a rate of 0.3 mV s −1 from −250 to +3500 mV relative to the OCP. Electrochemical impedance spectroscopy (EIS) or, AC measurements, were carried out applying a sinusoidal perturbation of 10 mV amplitude and a frequency sweep from 10 5 to 10 −2 Hz. The frequency response was analyzed using ZView software, the goodness of fit of the simulated spectra corresponded to chi-squared (square of the standard deviation between the original data and the calculated spectrum) values <0.01. The errors for the individual parameters of the equivalent electrical circuits (such as CPE and R ) were <5%.
2.6
Ion release analysis
The immersion test solutions were acidified with 200 μL of nitric acid (65 wt%) to dissolve precipitated titanium hydroxide. After filtering through a nylon filter with 22 μm pore size and 1:6 dilution in Millipore water, the samples were analyzed by inductively coupled plasma mass spectroscopy (ICP–MS) using an Agilent 7700 ICP–MS instrument equipped with concentric quartz nebulizer and Peltier-cooled nebulizing camera. The instrument was operated in standard mode, without using the reaction cell.
Argon was used as plasma maintaining carrier gas. ICP–MS operating conditions were as follows: forward power 1550 W, argon flow 0.99 L min −1 , nebulizer pump 0.3 rps.
Three titanium isotopes were measured simultaneously ( 47 Ti, 48 Ti and 49 Ti). Calibrations based on 47 Ti were made using both deionized water and 1:6 diluted SBF-blank solution in the range from 0 to 50 μg L −1 . The calibration curve was corrected with respect to a background level of Ti detected in blank SBF (22.7 ppb). The other two isotopes reflected to a greater ( 48 Ti) or lesser ( 49 Ti) extent the effects of the SBF-matrix. The results are cited as an average of two measurements with the errors representing the deviation of the maximum and minimum from the mean.
3
Results and discussion
3.1
Coating morphology and microstructure
Fig. 1 shows the surface morphologies of PEO1 and PEO2 coatings. The higher magnification images of the surfaces (insets) reveal submicron-size morphological differences in the superficial deposits originating from the electrolytes. Local EDX analysis, conducted in different areas of the coating surface, revealed that the respective surface compositions of each coating were fairly uniform. The average Ca/P surface ratio was 1.5 and 1.7 for PEO1-90 s and -600 s, respectively, which is close to stoichiometric hydroxyapatite (HA). PEO2-90 s contained similar absolute amounts of Ca and P to PEO1-90 s with an average Ca/P of ∼2, whereas PEO2-600 s showed ∼4 and ∼2 times lower amount of Ca and P, respectively, compared with PEO1-600 s, and its average Ca/P ratio was ∼4 ( Table 3 ).
Material | Elements (at%) | |||||||
---|---|---|---|---|---|---|---|---|
O | P | Ca | Ti | Fe | Ca/P | Ca/Ti | P/Ti | |
Ti CP | – | – | – | 99.7 ± 0.5 | 0.3 ± 0.1 | – | – | – |
PEO1-90 s | 61.3 ± 7.5 | 2.4 ± 0.5 | 3.7 ± 1.3 | 32.6 ± 1.2 | – | 1.5 | 0.11 | 0.07 |
PEO2-90 s | 59.9 ± 8.9 | 2.3 ± 0.5 | 4.6 ± 1.2 | 33.2 ± 1.2 | – | 2 | 0.13 | 0.07 |
PEO1-600 s | 73.6 ± 8.9 | 6.5 ± 1.3 | 11.1 ± 2.7 | 8.5 ± 1.1 | – | 1.71 | 1.31 | 0.76 |
PEO2-600 s | 66.1 ± 4.6 | 1.5 ± 0.3 | 6.0 ± 1.7 | 26.4 ± 4.1 | – | 4.00 | 0.22 | 0.06 |
As shown in Table 4 , after 600 s of PEO treatment, both coatings exhibited similar microhardness values. PEO1 had slightly greater roughness than PEO2, increasing this difference for a longer PEO treatment time. The lower limit of pore size is similar for all the coatings. The pores arise at the sites of the discharges channels due to the gas evolution through the molten oxide material during the PEO process; the upper limit of the pore size increases with treatment time and is the largest (∼13 μm) for PEO1. The pore population density did not exhibit a clear dependency on the treatment time.
PEO1-90 s | PEO2-90 s | PEO1-600 s | PEO2-600 s | |
---|---|---|---|---|
Microhardness (HV) | – | – | 276.5 ± 5.2 | 270.1 ± 4.2 |
Roughness (μm) | R a = 0.91 ± 0.18 R z = 5.69 ± 1.0 | R a = 0.58 ± 0.11 R z = 3.55 ± 0.71 | R a = 1.63 ± 0.32 R z = 9.08 ± 1.81 | R a = 1.28 ± 0.26 R z = 6.5 ± 1.3 |
Pore size (μm) | 0.4–3 | 0.4–2 | 0.4–12.7 | 0.4–6.2 |
Pore population density (pore mm −2 ) | ∼45,000 | ∼100,000 | ∼50,000 | ∼40,000 |
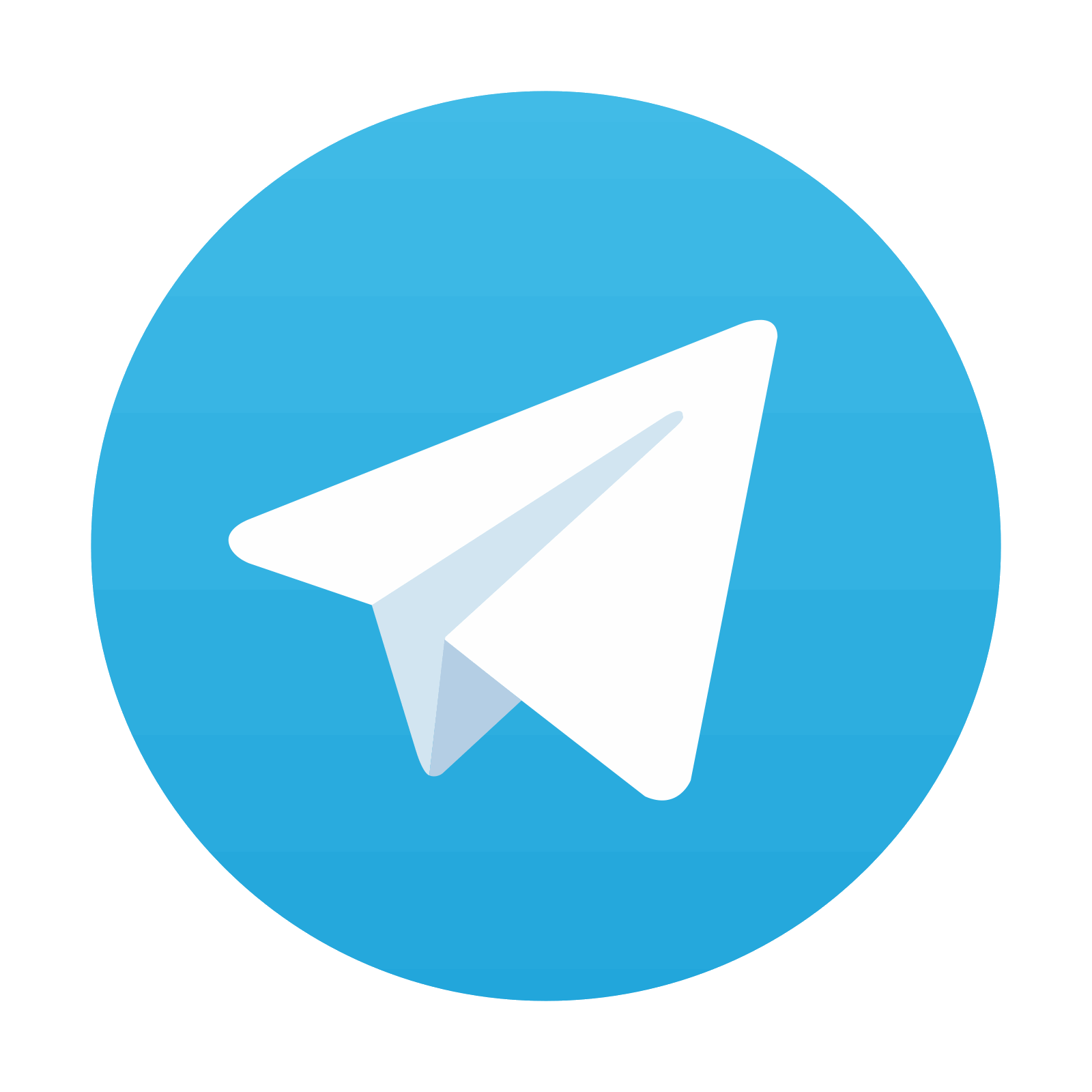
Stay updated, free dental videos. Join our Telegram channel

VIDEdental - Online dental courses
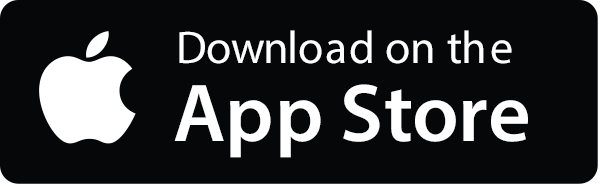
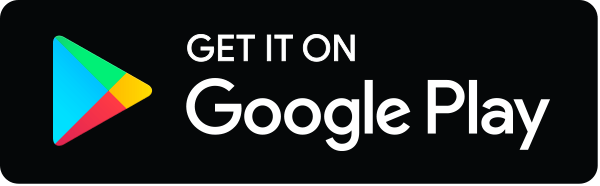
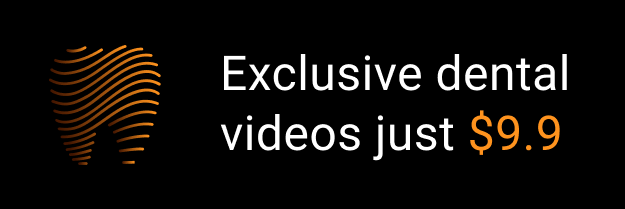