Abstract
Objective
To evaluate the reinforcement efficacy of hydroxyapatite (HA) whiskers in bis-GMA-based dental restorative composites and determine the effect of volume fraction on the mechanical properties.
Methods
Silanized HA whiskers and nano-scale powder were mixed in various proportions with bis-phenol A-glycidyl methacrylate (bis-GMA)-based polymer pastes. Equal parts of initiator and accelerator pastes were then mixed by hand. After curing at 25 ± 2 °C and storage in distilled water at 37 °C for 24 h, elastic modulus, fracture strength and work-to-failure in three-point bending, fracture toughness using a notchless triangular prism fracture method, and Vickers hardness were determined. Data were examined by means of one-way analysis of variance and linear regression.
Results
Reinforcing efficacy was significantly dependent on filler morphology. Whiskers had good dispersibility and wettability with bis-GMA-based polymer, conferring good reinforcement and toughening, significantly better than did the HA nano-scale powder.
Significance
HA whiskers provided better mechanical properties in bis-GMA-based composites compared with the nano-scale powder. Such whisker-reinforced materials may be beneficial compared with currently used dental restorative materials.
1
Introduction
Polymer-matrix composites are the most widely used dental restorative materials, whether after caries, fracture, or endodontic therapy, given their physico-mechanical properties . Among a wide range of such materials, those based on bis-phenol A-glycidyl methacrylate (bis-GMA) and related moieties are the most commonly used, having relatively low thermal expansion and shrinkage, low volatility, and clinically acceptable handling properties . However, their durability and strength are considered insufficient, and they lack bioactivity and biocompatibility. Although considerable efforts have been devoted to the improvement of the mechanical and biological properties through improvements in filler packing, optimization of filler content, and development of hybrid fillers, undesirable properties remain: inadequate strength, high failure rate, poor abrasion resistance, poor marginal adaptation, and lack of both adhesion to dentin and caries-protective properties, which deficiencies greatly hinder their use in large stress-bearing restorations . Thus, clinical application is mainly limited to the anterior region and small restorations in the posterior region.
Reinforcement by fibers or whiskers has been considered an effective way to improve the mechanical properties and reliability of biomedical composites due to their unusually high tensile strength and other remarkable characteristics , and fiber- or whisker-reinforced composites have attracted a great deal of attention in dentistry . Short fibers have given improved handling and better mechanical properties . However, residual stress in the matrix caused by polymerization shrinkage around large fibers was found to result in separation of those fibers from the matrix . Significant improvements are needed to extend the use of composites to stress-bearing applications such as direct posterior restorations and indirect crown and multiple-unit restorations. Xu et al. have used single-crystal ceramic whiskers treated by fusing silicate glass particles onto their surface for bis-GMA – triethylene glycol dimethacrylate (TEGDMA)-based composites. As would be expected, the mechanical properties were affected by the type of whisker and their length and volume fraction. Their flexural strength and fracture toughness were twice those of glass particle-reinforced composites. Unfortunately, most available fibers or whiskers, such as carbon, ceramic, glass, metal, and polymer, are at best bio-inert and could give rise to biocompatibility and bioactivity problems . In an attempt to address these issues, calcium phosphate cement powders or nanoscopic anhydrous dicalcium phosphate (DCPA) have been used together with silicon carbide whiskers to reinforce bis-GMA resin, which was said to produce non-cytotoxic composites with both strength and caries-inhibitory capability . The dark color of these whiskers remains a problem in practice.
With a view to improving the biological performance of the composites, bioactive particulate fillers, such as hydroxyapatite (Ca 10 (PO 4 ) 6 (OH) 2 , HA), amorphous calcium phosphate, and apatite–wollastonite glass–ceramic particles, have also been tried, with modest improvements in strength but showing good bonding ability to bone directly through a Ca–P-rich layer . The use of HA in restorative dentistry offers intrinsic radio-opacity, closer to ideal hardness ( i.e. similar to that of natural teeth), and therefore improved wear behavior , although they remained brittle and had a high failure rate in large stress-bearing restorations .
Thus, on the above basis, the most promising approach seems to be to use calcium phosphate-based whiskers or fibers, so that the development of HA whisker-reinforced dental restorative composites might be expected to be beneficial compared with currently used dental restorative materials. In fact, HA whisker-reinforced high-density polyethylene (HDPE) has shown better mechanical properties in comparison with those reinforced with spherical-particle HA . Unfortunately, HA whiskers prepared by commonly used methods are short, having a low aspect ratio, and with poor dispersibility due to entanglement or agglomeration, reducing their reinforcing efficacy . Moreover, to the best of our knowledge, no report has focused on bioactive HA whisker-reinforced bis-GMA-based dental restorative composites. In previous work, we have produced long and uniform HA whiskers with high aspect ratio . Therefore, the aim of this present work was to study the mechanical properties of bis-GMA-based composites reinforced with long HA whiskers with high aspect ratio with a view to developing novel, bioactive and strong dental restorative composites with high reliability.
2
Experimental procedure
2.1
Materials
HA whiskers (wHA) were prepared by hydrothermal homogeneous precipitation at 180 °C for 10 h, as previously described . Their length and aspect ratio were 116 ± 23 μm and 102 ± 32, respectively. A control particulate HA filler was prepared by an optimized wet chemical precipitation method , using 0.5 mol/L Ca(NO 3 ) 2 (AnalaR, BDH, Poole, England) and 0.3 mol/L (NH 4 ) 2 HPO 4 (AnalaR, BDH) solutions. After calcination in air at 800 °C for 2 h, the powder was sieved through a 180 mesh screen. This material had irregular morphology, being aggregates (5–20 μm) of rod-like crystals averaging 278 ± 65 nm in length and 52 ± 8 nm in diameter.
10 g portions of wHA or the aggregated nano-scale powder (anHA) were silanized by stirring into 125 mL silanization solution consisting of 10 mass% γ-methacryloyloxypropyltrimethoxy silane (γ-MPS) coupling agent and 2 mass% n -propylamine in cyclohexane (all Sigma–Aldrich, Milwaukee, WI, USA) under continuous stirring using a PTFE (polytetrafluoroethylene)-coated magnetic bar for 60 min . They were then heated to 90 °C (with continued stirring while still fluid) until the cyclohexane evaporated completely, and heated to 120 °C under vacuum for 2 h. After cooling under vacuum naturally to laboratory temperature (25 ± 2 °C), the mixture was washed with dichloromethane to remove residual γ-MPS and weakly attached material, filtered and re-dried at 120 °C in air for 24 h.
To obtain appropriate handling properties and degree of conversion, 25 mass% TEGDMA and 25 mass% hydroxyethyl methacrylate (HEMA) (all Sigma–Aldrich, USA) were used as diluents and co-monomers, spatulation-mixed with 50 mass% bis-GMA to form the resin matrix paste . Benzoyl peroxide (BPO) (98%, Riedel-deHaen, Seelze, Germany) and N,N-dihydroxyethyl-p-toluidine (DHEPT) (99%, Aldrich) were used as initiator and accelerator for the polymerization.
2.2
Preparation of HA-filled composites
The reactive resin components were prepared by adding 0.9 mass% of BPO and 0.9 mass% DHEPT to separate portions of the above resin, and stirring with a PTFE-coated magnetic stirring bar at 300 rpm until they had dissolved completely, forming initiator and accelerator pastes . Silanized filler to ∼4–30 vol% (6 values) was mixed into each matrix paste using a magnetic stirrer at ∼300 rpm for 24 h. Before the fabrication of composites, the pastes were exposed to a reduced pressure of ∼0.5 atm for ∼1 min to reduce the amount of air that had been entrained during mixing. Subsequently, equal masses of the two pastes were hand-mixed using a stainless steel spatula for about 1 min, and then placed in various molds (see Section 2.3 ), avoiding bubble entrapment, and covered with a polyethylene separating sheet (Dentsply, Dreieich, Germany). They were allowed to cure under a 100 N load in a leveling press (Leitz, Wetzlar, Germany) for 10–15 min before gentle demolding. One hour later, the specimens were hand-ground and polished with silicon carbide paper (220- to 1200-grit) under running water to remove excess material extruded from the mold. Each specimen was stored in distilled water at 37 °C for 24 h prior to mechanical testing. Unfilled resin was used as a control. The whole process was conducted at laboratory temperature (25 ± 2 °C) and relative humidity (55 ± 5%).
2.3
Characterization
Groups of six rectangular bar specimens (1.0 mm × 1.5 mm × 25 mm) were tested in three-point bending, span 10 mm, crosshead speed 1 mm/min, using a universal testing machine (Model 1185, Instron, High Wycombe, England) for flexural strength (FS). Elastic modulus ( E ) and work-to-fracture ( W f ) were calculated from the linear portion of and area beneath the recorded load–deflection curve, respectively. Fracture toughness ( K Ic ) was determined using a notchless triangular prism fracture method , for which groups of six equilateral triangular prism specimens, side 6.0 mm, length 12.0 mm, were used. Vickers hardness ( H V ) was determined on groups of ten disc-shaped specimens, diameter 10 mm, thickness 3 mm, under 50 g load for 10 s, using a micro-hardness tester (Orthoplan, Leitz, Germany).
The HA fillers were characterized by scanning electron microscopy (SEM) (XL30CP, Philips Electron Optics, Eindhoven, The Netherlands), X-ray powder diffraction (XRD) (X’Pert Pro, PANalytical BV, Almelo, The Netherlands) and Fourier-transform infrared spectroscopy (FTIR) (FTS-165, Bio-Rad Inc., Hercules, CA, USA). Fracture surfaces of composites were observed under a field emission-scanning electron microscope (FE-SEM) (LEO 1530, Oxford Instruments, Abingdon, UK). One-way analyses of variance and linear regressions were done in software (SigmaPlot, SYSTAT Software. San Jose, CA, USA; and GraphPad Prism5, GraphPad Software, La Jolla, CA, USA).
2
Experimental procedure
2.1
Materials
HA whiskers (wHA) were prepared by hydrothermal homogeneous precipitation at 180 °C for 10 h, as previously described . Their length and aspect ratio were 116 ± 23 μm and 102 ± 32, respectively. A control particulate HA filler was prepared by an optimized wet chemical precipitation method , using 0.5 mol/L Ca(NO 3 ) 2 (AnalaR, BDH, Poole, England) and 0.3 mol/L (NH 4 ) 2 HPO 4 (AnalaR, BDH) solutions. After calcination in air at 800 °C for 2 h, the powder was sieved through a 180 mesh screen. This material had irregular morphology, being aggregates (5–20 μm) of rod-like crystals averaging 278 ± 65 nm in length and 52 ± 8 nm in diameter.
10 g portions of wHA or the aggregated nano-scale powder (anHA) were silanized by stirring into 125 mL silanization solution consisting of 10 mass% γ-methacryloyloxypropyltrimethoxy silane (γ-MPS) coupling agent and 2 mass% n -propylamine in cyclohexane (all Sigma–Aldrich, Milwaukee, WI, USA) under continuous stirring using a PTFE (polytetrafluoroethylene)-coated magnetic bar for 60 min . They were then heated to 90 °C (with continued stirring while still fluid) until the cyclohexane evaporated completely, and heated to 120 °C under vacuum for 2 h. After cooling under vacuum naturally to laboratory temperature (25 ± 2 °C), the mixture was washed with dichloromethane to remove residual γ-MPS and weakly attached material, filtered and re-dried at 120 °C in air for 24 h.
To obtain appropriate handling properties and degree of conversion, 25 mass% TEGDMA and 25 mass% hydroxyethyl methacrylate (HEMA) (all Sigma–Aldrich, USA) were used as diluents and co-monomers, spatulation-mixed with 50 mass% bis-GMA to form the resin matrix paste . Benzoyl peroxide (BPO) (98%, Riedel-deHaen, Seelze, Germany) and N,N-dihydroxyethyl-p-toluidine (DHEPT) (99%, Aldrich) were used as initiator and accelerator for the polymerization.
2.2
Preparation of HA-filled composites
The reactive resin components were prepared by adding 0.9 mass% of BPO and 0.9 mass% DHEPT to separate portions of the above resin, and stirring with a PTFE-coated magnetic stirring bar at 300 rpm until they had dissolved completely, forming initiator and accelerator pastes . Silanized filler to ∼4–30 vol% (6 values) was mixed into each matrix paste using a magnetic stirrer at ∼300 rpm for 24 h. Before the fabrication of composites, the pastes were exposed to a reduced pressure of ∼0.5 atm for ∼1 min to reduce the amount of air that had been entrained during mixing. Subsequently, equal masses of the two pastes were hand-mixed using a stainless steel spatula for about 1 min, and then placed in various molds (see Section 2.3 ), avoiding bubble entrapment, and covered with a polyethylene separating sheet (Dentsply, Dreieich, Germany). They were allowed to cure under a 100 N load in a leveling press (Leitz, Wetzlar, Germany) for 10–15 min before gentle demolding. One hour later, the specimens were hand-ground and polished with silicon carbide paper (220- to 1200-grit) under running water to remove excess material extruded from the mold. Each specimen was stored in distilled water at 37 °C for 24 h prior to mechanical testing. Unfilled resin was used as a control. The whole process was conducted at laboratory temperature (25 ± 2 °C) and relative humidity (55 ± 5%).
2.3
Characterization
Groups of six rectangular bar specimens (1.0 mm × 1.5 mm × 25 mm) were tested in three-point bending, span 10 mm, crosshead speed 1 mm/min, using a universal testing machine (Model 1185, Instron, High Wycombe, England) for flexural strength (FS). Elastic modulus ( E ) and work-to-fracture ( W f ) were calculated from the linear portion of and area beneath the recorded load–deflection curve, respectively. Fracture toughness ( K Ic ) was determined using a notchless triangular prism fracture method , for which groups of six equilateral triangular prism specimens, side 6.0 mm, length 12.0 mm, were used. Vickers hardness ( H V ) was determined on groups of ten disc-shaped specimens, diameter 10 mm, thickness 3 mm, under 50 g load for 10 s, using a micro-hardness tester (Orthoplan, Leitz, Germany).
The HA fillers were characterized by scanning electron microscopy (SEM) (XL30CP, Philips Electron Optics, Eindhoven, The Netherlands), X-ray powder diffraction (XRD) (X’Pert Pro, PANalytical BV, Almelo, The Netherlands) and Fourier-transform infrared spectroscopy (FTIR) (FTS-165, Bio-Rad Inc., Hercules, CA, USA). Fracture surfaces of composites were observed under a field emission-scanning electron microscope (FE-SEM) (LEO 1530, Oxford Instruments, Abingdon, UK). One-way analyses of variance and linear regressions were done in software (SigmaPlot, SYSTAT Software. San Jose, CA, USA; and GraphPad Prism5, GraphPad Software, La Jolla, CA, USA).
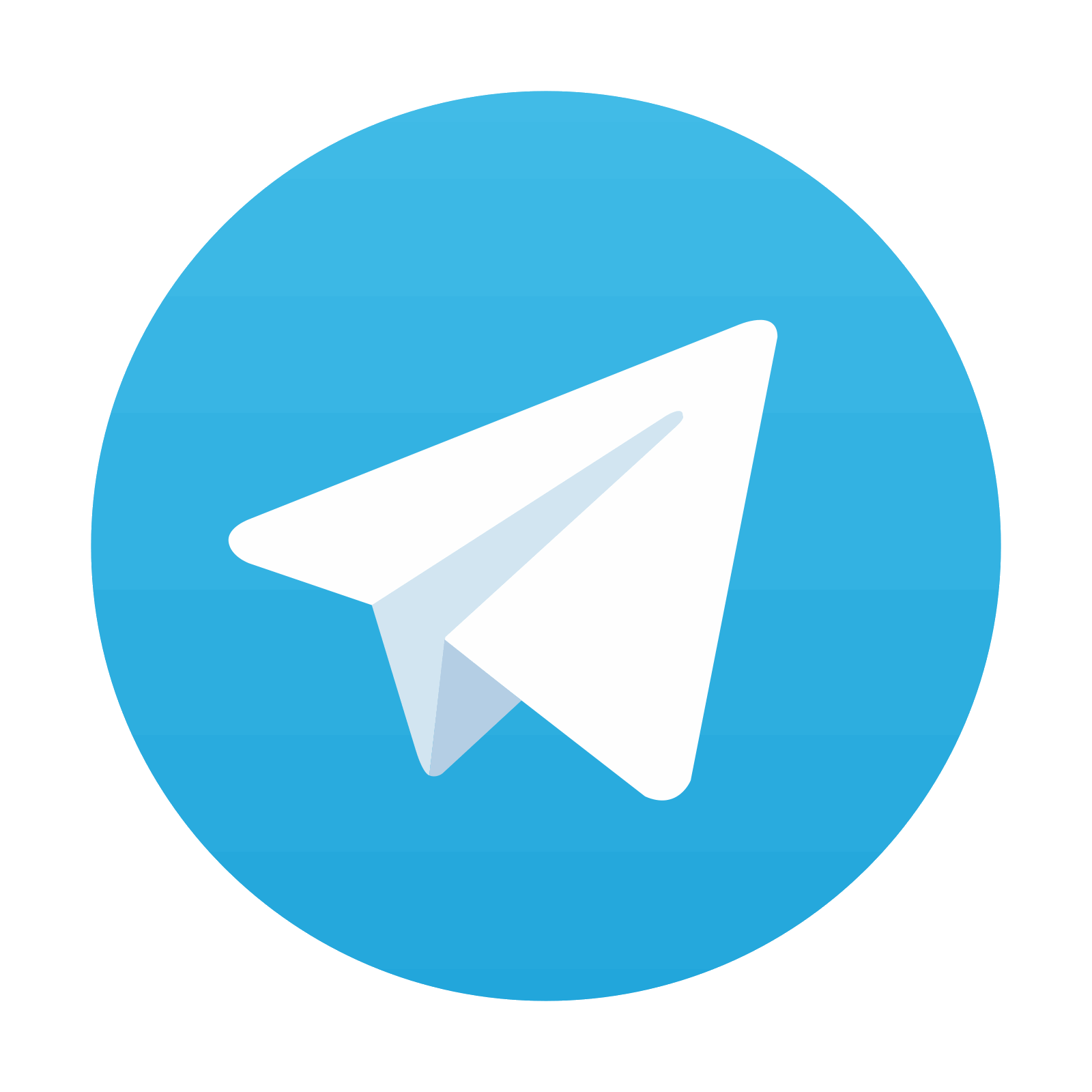
Stay updated, free dental videos. Join our Telegram channel

VIDEdental - Online dental courses
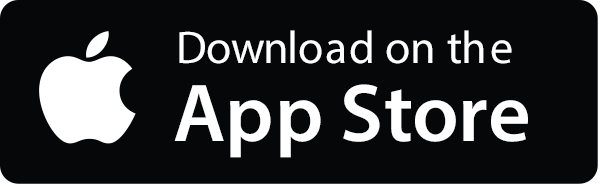
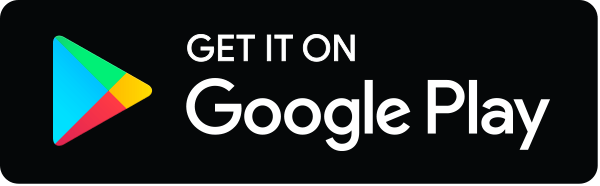