Highlights
- •
Replacing barium glass by DCPD did not affect composite degree of conversion.
- •
Replacing barium glass by DCPD reduced 24-h strength, but not elastic modulus.
- •
At 28 days, strength and modulus reductions were more severe in the presence of DCPD.
- •
DCPD increased K Ic after 24 h, but caused a reduction after 28 days.
- •
Calcium release was not affected by DCPD content. HPO 4 2− was marginally affected.
Abstract
Objective
To evaluate the effect of the replacement of barium glass by dicalcium phosphate dihydrate (DCPD) particles on the mechanical properties and degree of conversion (DC) of composites. Additionally, calcium and hydrogen phosphate (HPO 4 2− ) release were followed for 28 days.
Methods
Nine composites containing equal parts (in mols) of BisGMA and TEGDMA and 40, 50 or 60 vol% of total filler were manipulated. Filler phase was constituted by silanated barium glass and 0%, 10% or 20% of DCPD particles. DC was determined by near-FTIR. Biaxial flexural strength (BFS) and modulus ( E ) were tested using the “piston on three balls” method, while fracture toughness ( K Ic ) used the “single edge notched beam” method. Specimens were tested after 24 h and 28 days in water. Ion release was determined using inductively coupled plasma optical emission spectrometry (ICP-OES). Data were analyzed by ANOVA/Tukey (DC and ion release) or Kruskal–Wallis/Mann–Whitney (mechanical properties; alpha: 5%).
Results
DC was not affected by DCPD. The presence of DCPD reduced BFS for both storage times, while differences in E became evident after 28 days. After 24 h, K Ic increased with the addition of DCPD; after 28 days, however, K Ic decreased only for DCPD-containing composites. Calcium release was similar for both DCPD contents and remained fairly constant during the 28-day period. Overall, HPO 4 2− release was higher at 7 days and did not decrease after 14 days.
Significance
The composite with the highest filler level and 10% DCPD represented the best compromise between mechanical properties after aging in water and ion release.
1
Introduction
The development of permanent restorative materials with remineralizing or antimicrobial activity has been an aspiration in restorative dentistry as a possible path to reduce the risk of secondary caries , one of the main causes of restoration failure and replacement . The first attempts to incorporate calcium orthophosphate particles in dimethacrylate resins as ion source for remineralization date back several decades , but only recently orthodontic cements and sealants containing amorphous calcium phosphate (ACP) nanoparticles became available for clinical use .
Early on, the difficulties of combining high fracture strength with high levels of ion release became evident. Due to the lack of a chemical bond between the calcium phosphate particles and the resin phase, the former behave as large flaws (i.e. several micrometers in width), concentrating stress and acting as crack initiation sites. In fact, when 40% (by weight) of calcium phosphate particles were added to a dimethacrylate resin, its fracture strength was reduced by approximately 50% . Interestingly, elastic modulus is not influenced by the bond (or the lack of thereof) between resin and particle phases, as successive additions of tetracalcium phosphate (TTCP) particles to an unfilled resin led to cumulative increases in modulus .
Several attempts were made to improve the fracture strength of resin-based materials containing calcium phosphates. In broad terms, the tested approaches can be divided into two groups, namely, modification/functionalization of calcium phosphate particles and association of ion-releasing fillers with a reinforcing phase. The addition of the glass forming compounds tetraethoxysilane (TEOS) or zirconyl chloride in the synthesis of ACP resulted in 25–33% increase in the fracture strength of materials containing 40 wt% of filler, in comparison with those containing unmodified ACP particles . Hydroxyapatite particles functionalized with acrylic acid increased the flexural strength of a BisGMA/TEGDMA-based material by 75% in comparison to the use of non-functionalized particles . Finally, several studies tested the effect of silanization of calcium phosphate particles. Though these studies consistently reported higher fracture strengths for composites containing silanized calcium phosphate particles in comparison to unmodified particles , some of them also found that silanization hindered ion release .
The association of calcium phosphate particles with a reinforcing phase in resin-based materials involved the use of silanated, silica-fused silicon carbide or silicon nitride whiskers , or silanated glass particles . The use of whiskers as reinforcing phase in resin-based materials containing calcium phosphates was very effective from a mechanical standpoint . In fact, the flexural strength of composites containing equal mass fractions of calcium phosphate and whiskers matched that of a commercial hybrid composite with similar filler fraction, while their flexural modulus was actually higher . However, these materials have the drawback of being opaque and, therefore, had to be formulated as two-paste systems.
The weakening effect of calcium phosphate particles on resins can be exemplified by a study reporting that 20–30 wt% of silanated barium glass had to be added to a resin matrix containing 40 wt% TTCP in order to bring its strength to the level of the unfilled resin . Only a few studies tested the effect of replacing glass fillers by calcium phosphate particles. For instance, the replacement of 15% of glass particles by ACP in experimental composites containing 75 wt% of filler led to increased wear , and a significant reduction in strength, but had no effect on elastic modulus . Another important issue is the effect of calcium phosphate particles on composite degradation over time. Available information in the literature suggests that prolonged water immersion had similar effects on composites containing only glass particles (75 wt%) or up to 20 wt% of ACP particles .
Considering the effectiveness of glass fillers as reinforcing phase for stress-bearing restorative composites, it is important to determine the percentage of glass fillers that could be replaced by calcium phosphate particles without jeopardizing the mechanical properties and, at the same time, ensuring enough ion release for remineralization of caries lesions. Based on the scant amount of information on the effects of replacing silanated glass fillers with bioactive particles, the objective of the present study was to evaluate how degree of conversion, mechanical properties and ion release of experimental composites were affected when dicalcium phosphate dihydrate (DCPD) particles were incorporated in the resin matrix at the expense of reinforcing fillers. Different total filler levels were tested, as well as the effect of prolonged aging in water. The null hypotheses were: (1) the replacement of silanated glass fillers by DCPD does not affect the degree of conversion or the mechanical properties, regardless of the total filler content or DCPD levels considered, (2) the effects of prolonged immersion in water on mechanical properties are not influenced by the total filler content or by the presence of DCPD, and (3) ion release is similar among total filler content and DCPD levels.
2
Method
2.1
Composite formulation
Nine composites were prepared, constituted by a 1:1 ratio, in mols, of bisphenol-A glycidyl dimethacrylate (BisGMA) and triethylene glycol dimethacrylate (TEGDMA), 0.5% (by weight) of camphorquinone and ethyl-4-dimethylamino benzoate (EDMAB, all components from Sigma-Aldrich, St. Louis, MO, USA). The experimental design was based on a factorial model with total filler level (40%, 50% or 60% by volume) and DCPD content (0%, 10% or 20% by volume) as main factors. Silanated barium glass ( D 50 : 0.5 μm) and proprietary DCPD particles ( D 50 : 8.0 μm, CaHPO 4 ·2H 2 O, Labsynth, Diadema, SP, Brazil) were used. Their filler size distributions were determined by laser light scattering (Mastersizer 2000, Malvern Instruments Ltd., Malvern, UK) and are shown in Fig. 1 . A transmission electron microscopy image of part of a nano-structured agglomerate is shown in Fig. 2 . Particle densities (barium glass: 2.4 g/cm 3 ; DCPD: 2.7 g/cm 3 ), necessary to calculate the amounts used in the tested formulations, were determined in a Helium picnometer (Ultrapyc 1200e, Quantachrome Instruments, Boynton Beach, FL, USA). Composites were mechanically mixed under vacuum (Speedmixer DAC 150.1 FVZ-K, FlackTek Inc., Landrum, SC, USA) and kept under refrigeration until 2 h before use.


2.2
Degree of conversion
Degree of conversion ( n = 3) was determined by Fourier-transformed near-infrared spectroscopy (near-FTIR). The unpolimerized material was placed in a silicone mold with 7 mm in diameter and 1 mm in thickness and both were pressed between two microscopy glass slides. The spectrum of the unpolimerized material was obtained between 4000 and 10,000 cm −1 by the co-addition of 32 scans with 4 cm −1 resolution (Vertex 70; Bruker Optik GmbH, Ettlingen, Germany). Then, the composite was photoactivated through the glass slide (Radii Cal, SDI, Bayswater, Australia), receiving 24 J/cm 2 (1200 mW/cm 2 for 20 s) and the whole setup was dry-stored at 37 °C. After 24 h, a new spectrum was obtained. Degree of conversion was calculated as the ratio between the areas of the absorption band located at 6165 cm −1 (corresponding to the absorption of the C–H 2 group) of the polymerized and the unpolimerized material.
2.3
Biaxial flexural test
Disk-shaped specimens (12 mm × 1.2 mm, n = 20) were built using a stainless steel split mold. Photoactivation was performed by overlapping four 10-s irradiations, one per quadrant. Half of the specimens were stored in water at 37 °C for 24 h, while the other half was stored for 28 days. After storage, the specimens were placed on a “piston on three balls” setup positioned on a universal testing machine (model 5565, Instron Corp., Norwood, MA, USA). In this setup, the specimen was supported by three 2.5-mm diameter steel spheres distributed on a 10-mm diameter circumference 120° apart from each other, and the load was applied by a flat piston with 1.2 mm in diameter. The discs were positioned in a way that the irradiated surface would be under compressive stresses and fractured at a cross-head speed of 0.5 mm/min. Deflection at the center of the specimen was monitored by a deflectometer (model W-E401-E, Instron Corp, USA). Biaxial flexural strength (BFS) in MPa, was calculated according to the following equations:
BFS = − 0.2387 P ( X − Y ) b 2
X = ( 1 + v ) ln r 2 r 3 2 + 1 − v 2 r 2 r 3 2
Y = ( 1 − v ) 1 − ln r 1 r 2 2 + 1 − v r 1 r 3 2
where P is the fracture load (in Newtons); b is the specimen thickness (in mm); v Poisson’s ratio; r 1 is the radius of the supporting spheres circumference (5.0 mm); r 2 is the radius of the loading piston (0.6 mm); r 3 is the specimen radius (in mm). A Poisson’s ratio of 0.3 was adopted for all composites .
Flexural modulus was calculated according to the following equation:
E = β P α 2 ω h 3 × 0.001
where E is the flexural modulus (in GPa), β is a constant related to the deflection at the center of the disk (0.509), P is the load (in Newtons), α is the disk radius (in mm), ω is the deflection corresponding to P and h is the disk thickness (in mm). Surface fractures were gold-sputtered and observed under scanning electron microscopy (LEO 430, LEO Electron Microscopy Ltd., Cambridge, UK).
2.4
Fracture toughness
Fracture toughness ( K Ic ) was determined using the “single-edge notched beam” method (SENB). Specimens were built using a stainless steel split mold (25.0 mm in length, 5.0 mm in width and 2.8 mm in thickness, n = 20) with a razor blade inserted to produce a sharp notch at mid-point of specimen’s length. The composite was covered by a mylar strip and photoactivated by three adjacent exposures of 40 s (Radii-Cal, SDI). After being stored in distilled water at 37 °C for 24 h or 28 days, specimens were tested in three-point bending (span: 20 mm) in a universal testing machine (model 5565, Instron Corp.) at a cross-head speed of 0.13 mm/min, loading the notched surface in tension. The fractured surfaces were analyzed by a stereomicroscope with a CCD camera (model SZ61, Olympus, Tokyo, Japan) at 90× magnification. The width ( w ) and thickness ( b ) of the specimen, and the notch length ( a ) were measured using the ImageJ software (National Institute of Health, Bethesda, USA). Six notch length measurements (three on each fracture surface) were averaged and only the specimens with a / w ratio between 0.45 and 0.50 were used. K Ic , in MPa m 0.5 , was calculated using the following equations:
K Ic = P × S b × w 1.5 × f a w
where P is the fracture load (in Newtons) and S is the span (in meters). The f ( a / w ) value was calculated as follows:
f a w = q w 3 1.99 − ( a / w ) × 1 − ( a / w ) × 2.15 − 3.93 ( a / w ) + 2.7 ( a / w ) 2 2 × 1 + 2 ( a / w ) × 1 − ( a / w ) 3 / 2
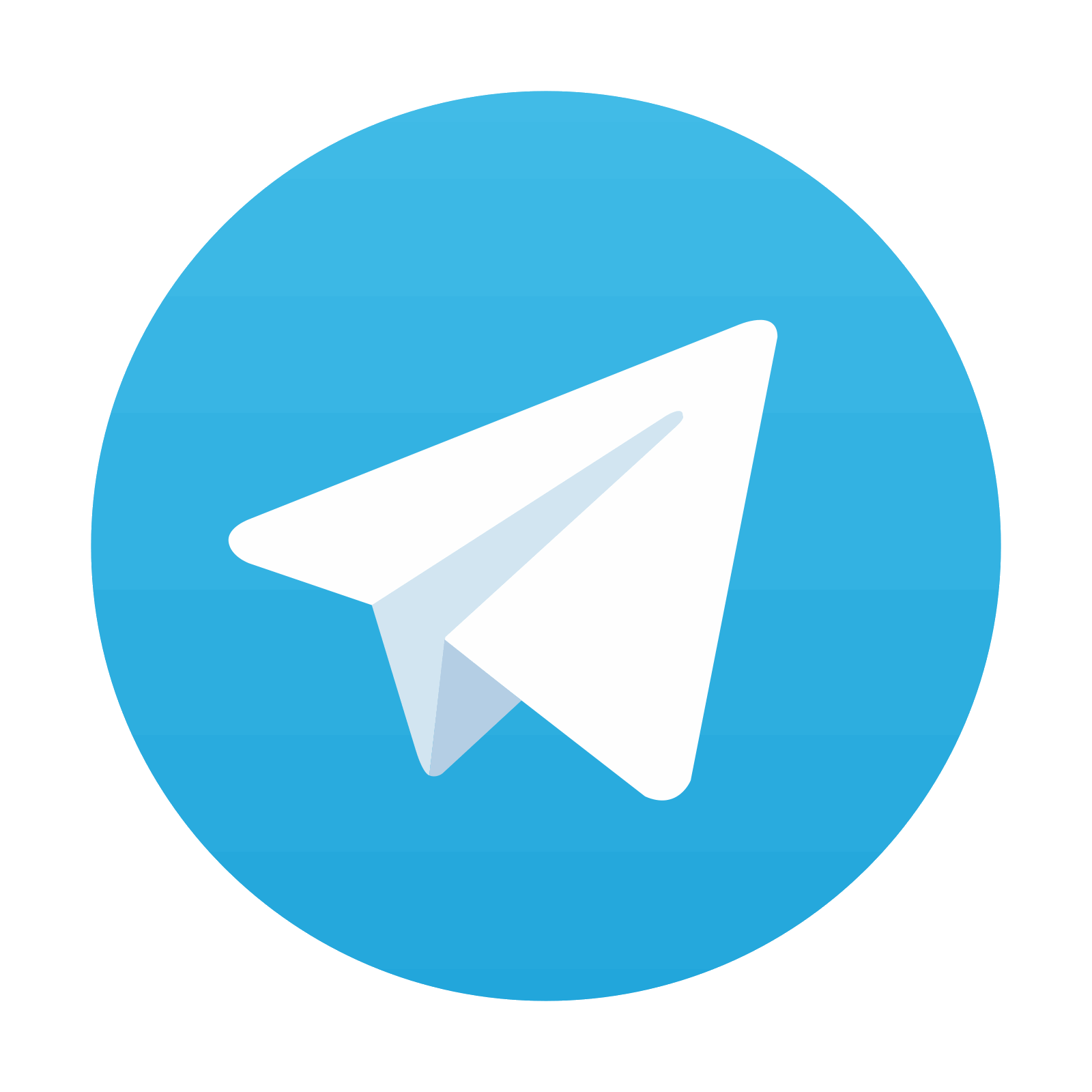
Stay updated, free dental videos. Join our Telegram channel

VIDEdental - Online dental courses
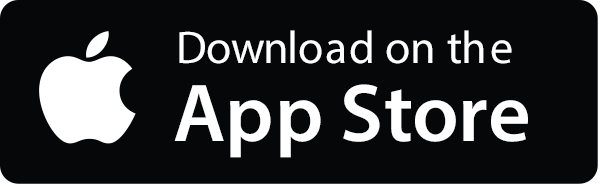
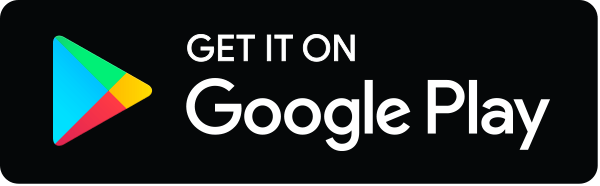