Abstract
Objectives
Materials that are capable of releasing ions such as calcium and fluoride, that are necessary for remineralization of dentin and enamel, have been the topic of intensive research for many years. The source of calcium has most often been some form of calcium phosphate, and that for fluoride has been one of several metal fluoride or hexafluorophosphate salts. Fluoride-containing bioactive glass (BAG) prepared by the sol–gel method acts as a single source of both calcium and fluoride ions in aqueous solutions. The objective of this investigation was to determine if BAG, when added to a composite formulation, can be used as a single source for calcium and fluoride ion release over an extended time period, and to determine if the BAG-containing composite can be recharged upon exposure to a solution of 5000 ppm fluoride.
Methods
BAG 61 (61% Si; 31% Ca; 4% P; 3% F; 1% B) and BAG 81 (81% Si; 11% Ca; 4% P; 3% F; 1% B) were synthesized by the sol–gel method. The composite used was composed of 50/50 Bis-GMA/TEGDMA, 0.8% EDMAB, 0.4% CQ, and 0.05% BHT, combined with a mixture of BAG (15%) and strontium glass (85%) to a total filler load of 72% by weight. Disks were prepared, allowed to age for 24 h, abraded, then placed into DI water. Calcium and fluoride release was measured by atomic absorption spectroscopy and fluoride ion selective electrode methods, respectively, after 2, 22, and 222 h. The composite samples were then soaked for 5 min in an aqueous 5000 ppm fluoride solution, after which calcium and fluoride release was again measured at 2, 22, and 222 h time points.
Results
Prior to fluoride recharge, release of fluoride ions was similar for the BAG 61 and BAG 81 composites after 2 h, and also similar after 22 h. At the four subsequent time points, one prior to, and three following fluoride recharge, the BAG 81 composite released significantly more fluoride ions ( p < 0.05). Both composites were recharged by exposure to 5000 ppm fluoride, although the BAG 81 composite was recharged more than the BAG 61 composite. The BAG 61 composite released substantially more calcium ions prior to fluoride recharge during each of the 2 and 22 h time periods. Thereafter, the release of calcium at the four subsequent time points was not significantly different ( p > 0.05) for the two composites.
Significance
These results show that, when added to a composite formulation, fluoride-containing bioactive glass made by the sol–gel route can function as a single source for both calcium and fluoride ions, and that the composite can be readily recharged with fluoride.
1
Introduction
Composites make up the largest percentage of materials used in restorative dentistry, largely because they have properties that make them both durable and esthetically pleasing. Recurrent caries is a major mode of failure in composite restorations and efforts are constantly being made to formulate materials that will decrease its occurrence. Materials incorporating potential remineralizing and antibacterial agents are examples.
Calcium is crucial for the restoration of damaged tooth tissue since it is a primary component of hydroxyapatite in enamel and dentin. When these tissues are damaged, a readily available source of calcium ions is necessary for remineralization. Calcium hydroxide has, for example, been used for many years in pulp-capping agents because of its function in remineralizing the surrounding tooth tissue . The resulting increase in pH, however, may cause irritation to surrounding soft tissue, thus limiting its applications. To overcome the negative effects of the hydroxide ion, calcium phosphates have been added to composite formulations as an alternative means to provide calcium ions .
Several clinical studies have demonstrated the cariostatic effects of fluoridated restorative materials. It has been reported that long-term exposure to fluoride with levels of 0.095–0.190 ppm fluoride in the saliva may be sufficient for cariostasis . Fluoride acts to reduce caries by being a biocide and by reducing the solubility of enamel and dentin through its incorporation into tooth tissue to form fluoroapatite . In addition, it has been shown that fluoride acts to remineralize damaged tooth tissue following demineralization . Furthermore, even though all fluoridated dental materials exhibit varying degrees of depletion of fluoride over time, even small amounts of fluoride provide some degree of cariostatic efficacy . For these reasons, a large number of restorative materials and oral care products contain fluoride ; these include glass ionomer cements, resin-modified glass ionomer cements, composites, adhesives, varnishes, toothpastes, and mouth rinses. Fluoride is commonly incorporated into these materials by the addition of either NaF, CaF 2 , SnF 2 , KPF 6 , YbF 3 , or fluoro-aluminosilicate glass. Because of their different solubilities, the rate of fluoride release and recharge for each substance is different . In vitro studies of the restorative materials have shown that there is an initial rapid release of fluoride due to surface dissolution, and a much slower diffusion-controlled release over time . These materials, therefore, serve as reservoirs of fluoride and can also be recharged with fluoride from a topical source . A problem, however, is that when these salts dissolve to release fluoride, they leave behind a void in the matrix .
Because carious lesions and further decay result from loss of calcium ions, it would be advantageous if a single additive to the composite formulation could act as a source of both fluoride and calcium ions. Bioactive glass (BAG) is such an additive; studies on BAG containing glass ionomer cements showed that BAG is a good source of both fluoride and calcium ions . This characteristic, therefore, sets BAG apart from the metal fluoride salts commonly used in dental materials since they release only fluoride. Bioactive glass made by the sol–gel process produces a material that has surface areas hundreds of times larger than BAG made by the melt-quench method . Because of its much greater surface area, sol–gel-derived BAG has the potential to more readily release these ions in composites, and be rechargeable when exposed to topical sources of calcium and fluoride. Furthermore, studies of composites formulated with BAG have shown that the mechanical properties are not significantly compromised by the addition of 12–15 wt% BAG , and the total material loss for a nonsilanated bioglass-containing composite was not quite 1% after 60 days in DI water .
In this study we test the hypothesis that the addition of fluoride-containing bioactive glass derived by the sol–gel method, will yield a material that releases both calcium and fluoride ions, and can be recharged with fluoride.
2
Materials and methods
2.1
Synthesis of fluoride-containing bioactive glass
Methoxyethanol (MeOEtOH) (Me = CH 3 ; Et = CH 2 CH 3 ), tetraethyl orthosilicate (Si(OEt) 4 ), triethyl phosphate (OP(OEt) 3 ), and calcium metal were purchased from Acrõs Organics; boron trifluoride etherate (BF 3 OEt 2 ) was purchased from Sigma–Aldrich. These reagents were used without further purification. Calcium methoxyethoxide (Ca(OEtOMe) 2 was synthesized immediately prior to use . In a typical reaction, stoichiometric quantities of Ca(OEtOMe) 2 , OP(OEt) 3 , and Si(OEt) 4 were combined under N 2 to yield a homogeneous solution. A solution (approx. 10%) of BF 3 OEt 2 in MeOEtOH was then added dropwise over 20 min to the vigorously stirred solution. The resulting solution was stirred under N 2 for 1 h, after which it was placed in an incubator at 37 °C and 100% humidity. A monolith formed after about 7 d; this was followed by the addition of DI water to insure complete hydrolysis. After another 3 d, the H 2 O and excess MeOEtOH were decanted and the gel was rinsed with 100% EtOH. The gel was then heated to 600 °C in a muffle furnace (Thermo Scientific, series 650–750) whereupon small pieces of colorless transparent glass formed. This glass was ball-milled in 100% EtOH to yield a slurry which was then dried at 37 °C, sieved, and micronized (Sturtevant, Model OM2, Hanover, MA, USA) Average particle size ranged from 0.04 to 3.0 μm, as determined by laser particle size measurements (Beckman Coulter LS13 320, Brea, CA). In this way, the bioactive glasses BAG 81 (81% Si; 11% Ca; 4% P; 3% F 1% B) and BAG 61 (61% Si; 31% Ca; 4% P; 3% F; 1% B) (mol%) were obtained. Surface areas of 316 and 129 m 2 g −1 , respectively, were determined by the BET method , with the use of a Quantasorb Sorption System (Quantachrome Corp., model QS 9, Greenvale, N.Y.). The amorphous character of the glass was established by X-ray diffraction , and its bioactivity was established by ion release of Ca and F with the use of atomic absorption spectroscopy (Shimadzu, Japan) and fluoride ion specific electrode (Orion 9409BN), respectively, and by the formation of hydroxyapatite upon immersion in simulated body fluid (infrared analysis: Thermo Scientific, Nicolet 6700, USA).
2.2
Preparation of composites
Resins were produced with 50:50 BisGMA:TEGDMA (Esstech), 0.4% CQ (Sigma–Aldrich), 0.8% EDMAB (Acrõs Organics), and 0.05% BHT (Acrõs Organics). Fillers composed of 57% by weight irregular 1–3 μm (average size) silane-treated-strontium glass (Bisco) mixed with 15 wt% fluoride-containing bioactive glass were added to the resin to obtain a total loading of 72 wt% filler. Control composites of identical composition contained aerosol silica filler (OX-50, Degussa) instead of BAG. The composite samples were packed into molds (4 mm diameter × 2 mm thick), light-cured (Demi, Kerr: 573 mW/cm 2 ) for 40 s, lightly abraded (4000 grit paper) on the top and bottom surfaces, and stored dry for 24 h prior to use.
2.3
Ion release from composites
Two disks in each of five vials, n = 5, were immersed in 2 mL DI water. These five samples were used for all subsequent fluoride and calcium measurements. Ion releases were measured at 2, 22, and 222 h time points prior to fluoride recharge, and at 2, 22, and 222 h time points following fluoride recharge. The disks were removed after each time period, rinsed with DI water, and re-immersed in fresh DI water.
2.3.1
Fluoride release
Supernatants (1 mL) after each time period were combined with 1 mL low-level TISAB (Orion) and fluoride concentrations measured with a fluoride ion specific electrode (Orion 9409BN). The disks were then placed in a 5000 ppm fluoride (NaF, Baker) solution for 5 min, rinsed and re-immersed in DI water. Fluoride was re-measured after 2, 22, and 222 h. All reported concentrations of the fluoride released were obtained by subtracting any contribution due to the control from the experimentally measured values. Average rates of release were calculated by dividing the fluoride measured at each time point by the time interval (2, 20, and 200 h) over which it was released.
2.3.2
Calcium release
After each time period as described above, 0.50 mL of the supernatant was combined with 4.5 mL of a freshly prepared 5% HNO 3 (Fisher Scientific) solution, and 0.50 mL of a solution made by reacting 5.803 g La 2 O 3 (Sigma–Aldrich) with 50.00 mL conc. HCl (Fisher Scientific) and diluting to 200 mL. The calcium ion concentration was determined by atomic absorption spectroscopy using standard methods .
2.4
Statistical analysis
The results for the average fluoride and calcium ion release per hour were analyzed by two-way analysis of variance (ANOVA) followed by Tukey’s post hoc comparison test ( p < 0.05).
2
Materials and methods
2.1
Synthesis of fluoride-containing bioactive glass
Methoxyethanol (MeOEtOH) (Me = CH 3 ; Et = CH 2 CH 3 ), tetraethyl orthosilicate (Si(OEt) 4 ), triethyl phosphate (OP(OEt) 3 ), and calcium metal were purchased from Acrõs Organics; boron trifluoride etherate (BF 3 OEt 2 ) was purchased from Sigma–Aldrich. These reagents were used without further purification. Calcium methoxyethoxide (Ca(OEtOMe) 2 was synthesized immediately prior to use . In a typical reaction, stoichiometric quantities of Ca(OEtOMe) 2 , OP(OEt) 3 , and Si(OEt) 4 were combined under N 2 to yield a homogeneous solution. A solution (approx. 10%) of BF 3 OEt 2 in MeOEtOH was then added dropwise over 20 min to the vigorously stirred solution. The resulting solution was stirred under N 2 for 1 h, after which it was placed in an incubator at 37 °C and 100% humidity. A monolith formed after about 7 d; this was followed by the addition of DI water to insure complete hydrolysis. After another 3 d, the H 2 O and excess MeOEtOH were decanted and the gel was rinsed with 100% EtOH. The gel was then heated to 600 °C in a muffle furnace (Thermo Scientific, series 650–750) whereupon small pieces of colorless transparent glass formed. This glass was ball-milled in 100% EtOH to yield a slurry which was then dried at 37 °C, sieved, and micronized (Sturtevant, Model OM2, Hanover, MA, USA) Average particle size ranged from 0.04 to 3.0 μm, as determined by laser particle size measurements (Beckman Coulter LS13 320, Brea, CA). In this way, the bioactive glasses BAG 81 (81% Si; 11% Ca; 4% P; 3% F 1% B) and BAG 61 (61% Si; 31% Ca; 4% P; 3% F; 1% B) (mol%) were obtained. Surface areas of 316 and 129 m 2 g −1 , respectively, were determined by the BET method , with the use of a Quantasorb Sorption System (Quantachrome Corp., model QS 9, Greenvale, N.Y.). The amorphous character of the glass was established by X-ray diffraction , and its bioactivity was established by ion release of Ca and F with the use of atomic absorption spectroscopy (Shimadzu, Japan) and fluoride ion specific electrode (Orion 9409BN), respectively, and by the formation of hydroxyapatite upon immersion in simulated body fluid (infrared analysis: Thermo Scientific, Nicolet 6700, USA).
2.2
Preparation of composites
Resins were produced with 50:50 BisGMA:TEGDMA (Esstech), 0.4% CQ (Sigma–Aldrich), 0.8% EDMAB (Acrõs Organics), and 0.05% BHT (Acrõs Organics). Fillers composed of 57% by weight irregular 1–3 μm (average size) silane-treated-strontium glass (Bisco) mixed with 15 wt% fluoride-containing bioactive glass were added to the resin to obtain a total loading of 72 wt% filler. Control composites of identical composition contained aerosol silica filler (OX-50, Degussa) instead of BAG. The composite samples were packed into molds (4 mm diameter × 2 mm thick), light-cured (Demi, Kerr: 573 mW/cm 2 ) for 40 s, lightly abraded (4000 grit paper) on the top and bottom surfaces, and stored dry for 24 h prior to use.
2.3
Ion release from composites
Two disks in each of five vials, n = 5, were immersed in 2 mL DI water. These five samples were used for all subsequent fluoride and calcium measurements. Ion releases were measured at 2, 22, and 222 h time points prior to fluoride recharge, and at 2, 22, and 222 h time points following fluoride recharge. The disks were removed after each time period, rinsed with DI water, and re-immersed in fresh DI water.
2.3.1
Fluoride release
Supernatants (1 mL) after each time period were combined with 1 mL low-level TISAB (Orion) and fluoride concentrations measured with a fluoride ion specific electrode (Orion 9409BN). The disks were then placed in a 5000 ppm fluoride (NaF, Baker) solution for 5 min, rinsed and re-immersed in DI water. Fluoride was re-measured after 2, 22, and 222 h. All reported concentrations of the fluoride released were obtained by subtracting any contribution due to the control from the experimentally measured values. Average rates of release were calculated by dividing the fluoride measured at each time point by the time interval (2, 20, and 200 h) over which it was released.
2.3.2
Calcium release
After each time period as described above, 0.50 mL of the supernatant was combined with 4.5 mL of a freshly prepared 5% HNO 3 (Fisher Scientific) solution, and 0.50 mL of a solution made by reacting 5.803 g La 2 O 3 (Sigma–Aldrich) with 50.00 mL conc. HCl (Fisher Scientific) and diluting to 200 mL. The calcium ion concentration was determined by atomic absorption spectroscopy using standard methods .
2.4
Statistical analysis
The results for the average fluoride and calcium ion release per hour were analyzed by two-way analysis of variance (ANOVA) followed by Tukey’s post hoc comparison test ( p < 0.05).
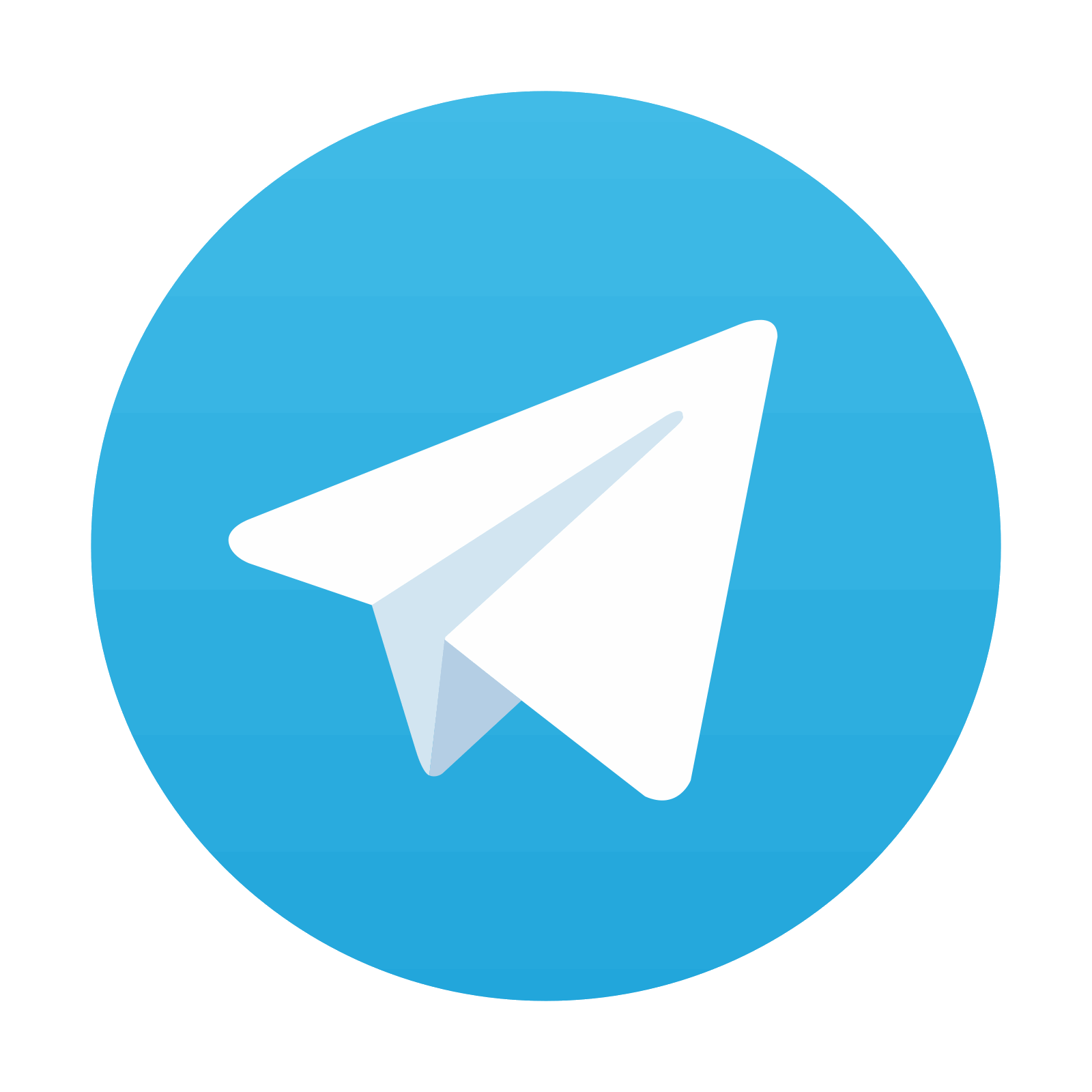
Stay updated, free dental videos. Join our Telegram channel

VIDEdental - Online dental courses
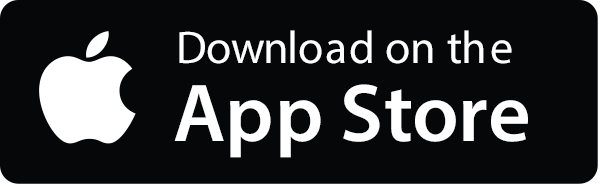
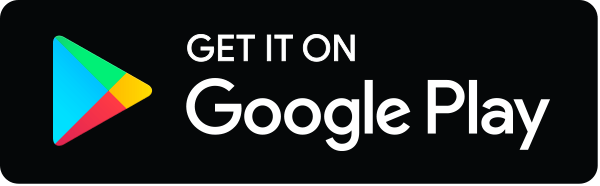