Introduction to pharmacology and therapeutics – pharmacokinetics
Alan Nimmo
Key Topics
- • Introduction to pharmacokinetics and the factors that affect drug concentration within the body
- • Drug diffusion and partitioning within the body
- • Elimination of drugs from the body – metabolism and excretion
- • Routes of drug administration
- • Quantifying drug kinetics
Learning Objectives
- • Be familiar with the factors that influence the concentration of a drug within the body
- • Be aware of factors, such as regional differences in pH, which may influence the distribution of drugs within the body
- • Be aware of the mechanisms involved in eliminating drugs from the body, and the potential for drug interactions and toxicity
- • Be familiar with the main routes of drug administration
- • Be aware of some of the basic approaches used to quantify drug kinetics
Introduction
While the science of pharmacodynamics helps explain how drugs produce their effects within the body, safe clinical practice is equally dependent upon a knowledge of pharmacokinetics. By definition, pharmacokinetics studies the movement of drugs, and in particular their ability to move from their site of administration to their site of action. However, it is also the science that determines the correct dose and route of administration of a particular drug in order to ensure that one achieves the required concentration of the drug at its target site.
The importance of correct dosing cannot be overstated. Drugs will only produce their beneficial effects within their ‘therapeutic range’. If the drug concentration is too low, then the required, beneficial effects will not be achieved, while if the dose is too high, unwanted or toxic effects of the drug will start to predominate. For some drugs, their therapeutic range may be quite wide, making them both easy and safe to use in clinical practice. However, other drugs may have a narrow therapeutic range, requiring careful management and monitoring in order to avoid adverse reactions. In the words of the Swiss-German physician Paracelsus (1493–1541), who has been credited as the founder of modern toxicology, ‘Solely the dose determines that a thing is not a poison’.
There is a whole range of factors that will influence the concentration of a drug at its target site and, in general, they are all interdependent. These factors are commonly divided into four components, referred to as the phases of drug disposition, these being absorption, distribution, metabolism and excretion, commonly abbreviated to ADME. When one thinks about administering a local anaesthetic in the dental clinic, one may feel that these factors are of little importance, since the drug is being ‘placed’ near the nerve you want to block. However, you will find it is much more difficult to achieve effective anaesthesia in an area where there is significant inflammation, as compared to non-inflamed tissue. One potential explanation for this lies in an understanding of basic pharmacokinetics.
Introduction to pharmacokinetics
While absorption is considered the first phase of drug disposition, it is perhaps worth considering the factors that affect drug distribution first of all, since these can have broad ramifications, including influencing the route of administration.
Distribution
For many drugs, following administration, they are transported around the body via the bloodstream. Drugs may be either injected directly into the circulation (i.e. intravenous injection), or they may enter the circulation, for example following absorption from the gastrointestinal tract. Once in the bloodstream, almost all drugs are transported in exactly the same way, by what is referred to as bulk-flow transfer, where the drug is transported rapidly around the body. Because most drugs are transported in the same way, this does not have a major influence on the pharmacokinetic characteristics of an individual drug. The one caveat to that is that within the bloodstream, many drugs will bind to the plasma proteins, which will influence their movement, but we will discuss this aspect separately. However, for a drug to produce its effects within the body, it will have to leave the bloodstream and diffuse to its target site. It is this ability of a drug to diffuse within the body that is a key factor in determining an individual drug’s pharmacokinetic properties.
In order to understand the way a drug is able to move around the body, perhaps the first thing that needs to be considered is the nature of the body itself. Our body does not consist of one single compartment, but instead is made up of a number of different compartments, each with its own physicochemical characteristics. The barriers that separate these various compartments are composed of our body’s cells, for example epithelial cells lining the gastrointestinal tract. With these cellular barriers, it is the phospholipid membrane of the cell that forms the actual barrier. For a drug to pass through such a barrier, there are two possibilities.
On the one hand, a drug may pass between the cells if there are gaps between neighbouring cells forming the barrier (paracellular movement). This is seen in many capillaries, where there are small pores, or intercellular clefts, between the vascular endothelial cells. These pores allow for the passage of small, water-soluble molecules through the barrier. However, larger molecules, such as plasma proteins, are too big to pass through these pores, and will be retained in the circulation.
However, other barriers, such as the blood-brain barrier, serve a protective function, and here there are tight junctions between neighbouring cells, giving the barrier more functional integrity. For a drug to cross such a barrier, it has to be able to pass through the cells (transcellular movement), rather than between them. The blood-brain barrier represents the most robust barrier within the body, with astrocyte foot processes providing an extra cell layer around the vascular endothelium to both restrict and regulate the movement of solutes.
There are four basic ways in which a drug molecule may diffuse through an epithelial or endothelial cell barrier. If the drug molecule is neither ionized nor polar, then the molecule will have sufficient lipid solubility to diffuse directly through the cell membrane. However, many drugs are either weak acids or bases, and hence, at any one time, exist in both an ionized and non-ionized form. The actual proportion of these forms, and therefore the overall lipid solubility of the drug, will depend upon the pH of the solution in which the drug is dissolved. We will revisit this concept in considering pH partitioning.
The second mechanism by which drugs can cross a cell membrane is in combination with a carrier protein that either facilitates diffusion, or enables active transport. Such carriers naturally transport endogenous chemicals, such as nutrients, but may also facilitate drug movement. These carriers are mainly confined to specific organs, such as the gastrointestinal tract and kidney, and are also important in blood-brain barrier function.
Thirdly, drugs can transverse the cell membrane by the process of pinocytosis, or ‘cell drinking’, where the cell membrane invaginates around the molecule forming a vesicle, which is then transported into the cell. Pinocytosis may assist larger molecules, such as insulin, to cross the blood-brain barrier, but is unlikely to assist the transport of small drug molecules.
Finally, the presence of aqueous pores (aquaporins) and ion channels may potentially enable the movement of very small molecules or ions, such as lithium, to enter the intracellular fluid. However, in general, lipid diffusion and carrier-mediated transport are the more important mechanisms for the transcellular movement of drugs.
Partitioning:
As mentioned, the body not only contains a variety of compartments, but those compartments have different physicochemical properties. For example, the plasma of the bloodstream is a protein-rich aqueous solution, while adipose tissue represents a predominantly lipid environment. The pH of the plasma in the bloodstream is very slightly alkaline (pH 7.4), while gastric acid makes the lumen of the stomach highly acidic (∼pH 2). These different chemical environments can lead to an uneven distribution of a drug within the body because, depending upon the chemical nature of a drug, it may have a greater affinity for one environment over another. The main factors that give rise to an uneven drug distribution within the body are pH differences across a barrier (pH partitioning), protein binding and sequestration into lipid.
pH Partitioning:
For most drugs to diffuse through the barriers between compartments within the body, they must be in a non-ionized, and hence lipid soluble form. However, because many drugs are weak acids or bases, they exist in both an ionized and non-ionized state. The proportion of the two forms will vary depending upon the pH of the solution in which it is dissolved. For example, if a weak acid is dissolved in an acidic solution, it will be predominantly non-ionized, as compared to when it is in a basic solution, where it will be mostly ionized. The converse is the case for a weak base, which will be predominantly ionized in an acidic solution. If one knows the dissociation constant, or pKa, of the drug in question, the precise proportion of the two forms can be calculated using the Henderson-Hasselbach equation. It is worth keeping in mind that the pKa does not indicate whether a drug is a weak acid or base, but rather indicates its tendency to dissociate, depending upon the pH of its environment.
pH partitioning occurs when one has solutions of different pH on either side of a barrier. Within the human body, this occurs most markedly between the lumen of the stomach and the bloodstream. Keeping in mind that only the non-ionized form of the drug can diffuse through the barrier, the concentration gradients that drive diffusion only relate to the non-ionized form. Hence, if you have a weak acid in an acidic environment, the concentration of the non-ionized form is high, while in an alkaline environment, the non-ionized form has a low concentration. This provides a driving force for diffusion. As a result, weak acids tend to move from a relatively acidic environment to a more alkaline one. The opposite happens with weak bases, where they tend to diffuse from a more alkaline environment to an acidic one.
From a dentistry perspective, pH partitioning may impact upon local anaesthesia when there is significant, local inflammation. By nature, local anaesthetics are weak bases. It is the ionized form of the anaesthetic that interacts with the binding site in the transmembrane pore of the voltage-gated Na+ ion channel to produce the blockade. Access to the channel’s binding site can occur either by direct diffusion through the neuronal membrane (hydrophobic pathway) or by first diffusing through the membrane into the cytoplasm, and then entering the active channel from the intracellular compartment (hydrophilic pathway). Both of these pathways rely on having a sufficient concentration of the non-ionized form of the anaesthetic outside the nerve, to drive diffusion through the neuronal membrane. Unfortunately, inflammation may cause the extracellular fluid to become more acid, reducing the concentration of the non-ionized form of the anaesthetic, thereby reducing diffusion of the anaesthetic into the nerve.
Protein binding:
In addition to acting on their target proteins, drugs may bind to other proteins, most notably the plasma protein, albumin. As a generalization, acidic drugs, such as NSAIDs, tend to bind more readily to plasma albumin than basic drugs, but there are a number of basic drugs, such as tricyclic antidepressants, that do bind. For some drugs, a significant proportion of the drug within the bloodstream may be bound to the plasma proteins (e.g. 99%), while only a small proportion is in free solution. This has a number of implications. Since the pores in the vascular endothelium are relatively small, plasma proteins, and with them the bound fraction of the drug, remain in the bloodstream, while only the drug in free solution is able to diffuse out of the circulation in order to produce its effects. For drugs with a high degree of protein binding, a significant proportion of the total drug within the body is retained in the circulation. In this way, plasma proteins can act as a ‘slow release’ mechanism for certain medications. The portion of a drug that is bound to the plasma proteins is also ‘protected’ from metabolism and excretion.
The other key aspect in relation to protein binding is it provides one mechanism by which drug–drug interactions can occur. Each albumin molecule has two drug-binding sites, and the concentration of albumin in the plasma is approximately 0.6 mmol/l. Given that most drugs produce their effects at relatively low concentrations (e.g. 1µM), there is an abundance of binding sites. However, for drugs that act at relatively high concentrations and exhibit a high degree of protein binding, there is the potential for competition for binding sites, and for one drug to displace another from plasma proteins. For drugs that are normally highly bound to plasma proteins, even the displacement of a relatively small proportion of the bound drug can have a huge impact on the concentration of the free drug, and with that, greatly increase the effects of the drug.
Sequestration into lipid:
Approximately 20% of our body mass is lipid. As indicated, many drugs need to be reasonably lipid soluble in order to reach their target sites within the body, and this is particularly true of drugs that are able to act on the CNS. As such, these drugs must have some tendency to enter the lipid compartments in the body. However, for most of these drugs, they are not so lipid soluble that there is any significant sequestration into body fat. The main exception to this is general anaesthetics, which rely on high lipid solubility for their anaesthetic potency. General anaesthetics will tend to accumulate in body fat, and this is responsible for both the slow onset of action, and slow recovery, that is seen with inhaled anaesthetics.
Elimination of drugs from the body
Most drugs are eliminated from the body by renal excretion. However, the kidneys are much more efficient at excreting water-soluble wastes as compared to lipid-soluble ones. As such, the body’s ability to excrete more lipophilic agents occurs in two stages. First, these drugs will undergo metabolic alteration in order to generate metabolites that are more water-soluble than the parent drug. These water-soluble metabolites can then be excreted by the kidneys.
Metabolism:
Drug metabolism predominantly occurs within the liver, although there are enzymes in other tissues, including the plasma, that have a metabolic potential. Depending upon the local anaesthetic used, both aspects of metabolism are important. Local anaesthetics that contain an ester bond, such as benzocaine, are rapidly metabolized by plasma esterases, while those containing an amide bond, such as lignocaine, are metabolized by hepatic amidases, giving them a longer half-life.
Drug metabolism primarily occurs as a two-step process, involving two types of metabolic reaction, referred to as Phase I and Phase II reactions. These two reactions occur in a sequential manner. Phase I reactions involve processes such as oxidation and reduction, which make the drug more chemically reactive, and sometimes more toxic. These are followed by a second set of reactions (Phase II), which involve conjugation. The addition of a chemical substituent on to the molecule renders the drug more water soluble, as well as normally abolishing activity.
While metabolism normally provides a mechanism for abolishing drug activity, there are a few drugs that are administered in an inactive form, and rely on metabolism to convert them into the active form, for example the corticosteroid, prednisone needs to be converted into its active form, prednisolone.
The other potential issue around drug metabolism is toxicity. Toxic metabolites may be generated by the Phase I reactions, but while they are rapidly ‘mopped-up’ by the Phase II reactions, they do not normally pose an issue. However, in certain situations, such as drug overdose, the toxic, Phase I metabolites may be generated more rapidly than they are removed, giving the potential to cause harm. Perhaps, not surprisingly, the liver is one of the main victims in this situation, since these toxic metabolites are at their highest concentration in the liver. This situation is epitomized with paracetamol, where the parent drug is a very safe agent. However, in an overdose situation, the hydroxylated metabolite builds up, potentially causing liver damage or failure.
Excretion:
Renal excretion is the main route of elimination of drugs from the body. However, there are significant differences in the way the kidneys handle different drugs.
Drugs in free solution (i.e. not bound to plasma proteins) are readily filtered in the kidneys, and enter the glomerular filtrate in the nephron. As the filtrate passes through the nephron its composition, including drug concentration, are markedly altered by the processes of tubular reabsorption and secretion. Some drugs, particularly those that are relatively lipid soluble, may diffuse from the renal tubule back into the circulation, resulting in slow renal excretion. In contrast, some drugs are actively secreted from the circulation into the forming urine in the renal tubules, resulting in rapid renal excretion. The antibiotic, penicillin, is particularly susceptible to this rapid renal clearance as a result of tubular secretion. However, this rapid elimination of penicillin can be prevented by formulating it with a drug, such as probenecid, that competes for the renal transport protein.
In addition to renal excretion, drugs may also be removed from the body by excretion into bile, and subsequent elimination in faeces, as well as by exhalation. Exhalation is the main route of elimination of gaseous, or volatile, anaesthetics, as well as the inhaled dental sedative, nitrous oxide.
Routes of administration and drug absorption
There are a number of different routes of administration available for medications, each with their own benefits and limitations. In general, the most popular route for drug administration is the oral route. However, the popularity of oral administration reflects patient preference and compliance, rather than the effectiveness of the route. Indeed, there are a whole range of factors that impact upon the absorption of drugs from the gastrointestinal (GI) tract.
Agents taken orally are subject to the acidic environment of the stomach, as well as the digestive enzymes found in the GI tract. The rate of transit of a drug through the gut, and hence rate of absorption, is affected by GI motility, which may be influenced by food intake, disease and other medications. Absorption from the gut is also influenced by splanchnic blood flow, as well as the potential for interactions with GI contents. For example, the absorption of tetracycline antibiotics can be inhibited by Ca2+ ions. However, perhaps the most significant issue with oral administration, is that substances absorbed from the GI tract enter the hepatic circulation, and travel to the liver prior to entering the systemic circulation. As mentioned, the liver is the main site of drug metabolism and, as a result, significant amounts of a drug may be metabolized before reaching the systemic circulation, giving rise to a phenomenon known as first-pass, or pre-systemic metabolism. While one can compensate for this metabolism by administering increased amounts of a drug, this leads to increased levels of metabolites, and potential unwanted effects.
Injection represents a more reliable route of administration. For systemic drug action, intravenous injection provides certainty over how much drug entered the systemic circulation and when. Other effective, but slower routes of systemic administration, are intramuscular and subcutaneous injections, where the drug is absorbed from the site of injection, into the circulation. The rate of absorption will depend upon factors such as local blood flow. Injections also provide a mechanism for topical, or local, effects, as with local anaesthesia in dentistry. The local actions of the anaesthetic may be enhanced by formulating it with a vasoconstrictor, such as adrenaline, in order to reduce local blood flow, and retain the anaesthetic at the site of administration.
Volatile agents, such as gaseous general anaesthetics and nitrous oxide, can be administered by inhalation. These gases can quickly equilibrate between alveolar air and arterial blood particularly if, like nitrous oxide, they have a low solubility. Drugs may also be absorbed through mucous membranes, enabling topical effects of agents within the oral cavity. However, sublingual and buccal administration may also be used for systemic administration, with the advantage that the drug is absorbed directly into the systemic circulation, by-passing pre-systemic metabolism. A similar effect may be achieved through rectal administration.
Basic kinetics
While the ability of a drug to get to its target site and start producing its beneficial effects is a key consideration, we do also have to consider how a drug is eliminated from the body. The dosing, and dosing regimen of a drug is determined by both the absorption of a drug by the body, and its subsequent elimination. A detailed consideration of drug kinetics is beyond the scope of this chapter, but we will introduce some key terms and concepts.
The rate at which a drug is eliminated from the body is normally expressed as the rate of clearance (CL). As mentioned, drugs may be cleared from the body by different routes, such as renal excretion or metabolism. The overall clearance of a drug (CLtot) represents the sum of the different routes of clearance, for example CLren + CLmet would account for elimination by both renal excretion and metabolism. The way in which clearance is expressed (ml/min or l/h) is best understood by thinking about renal clearance (CLren). The rate at which a drug is being eliminated by the kidneys is related to its concentration in the urine (Cu), and the volume of urine (Vu) that is produced in a particular time. This rate of elimination also needs to be related to the amount of drug in the body, reflected by its plasma concentration (Cp). The units of clearance, such as ml/min, reflect the volume of plasma that would be cleared of the drug in that particular time.

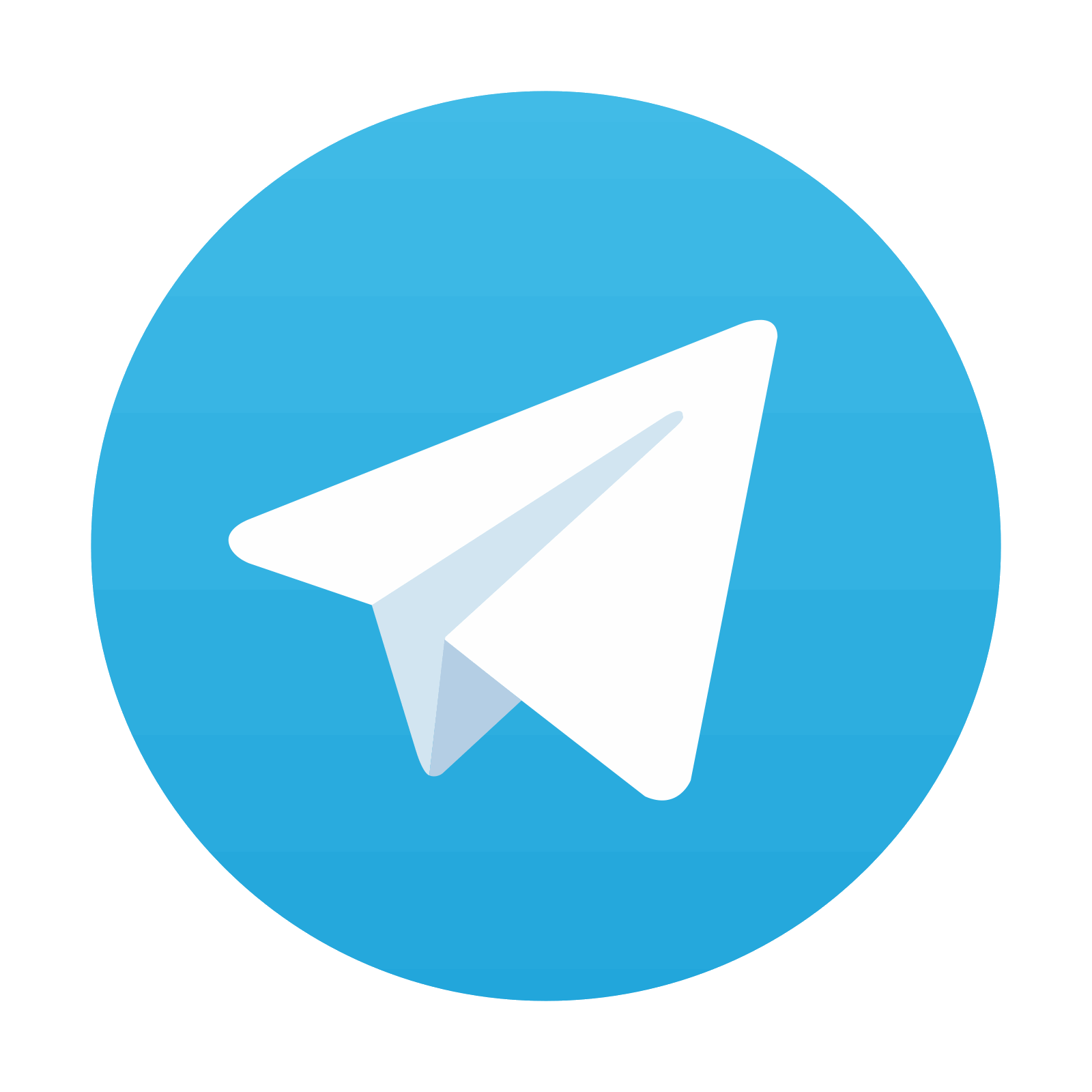
Stay updated, free dental videos. Join our Telegram channel

VIDEdental - Online dental courses
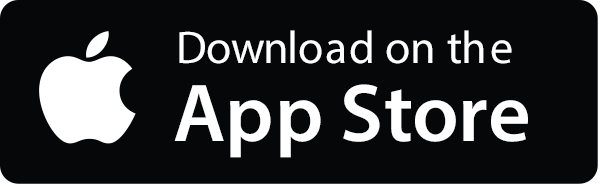
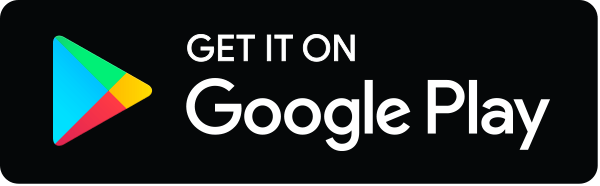