Key points
- •
The development and implementation of medical technology in orbital reconstructive surgery is a logical consequence of the philosophy to optimize the treatment procedure before, during, and after surgery in terms of maximum control for the surgeon and the aim for optimal outcome for the individual patient.
- •
During the surgical procedure, the optimal virtual surgical planning must be transferred to the surgical site as accurately as possible; for this purpose, a variety of different methods and devices may be used and even combined, depending on the complexity of the task.
- •
The adage that “the best way to predict the future is to invent it” (Alan Kay) has proved true for orbital reconstruction in the past and will be the key to future innovations.
Introduction: the rationale of technology in orbital surgery
Over the last 20 years, rapid changes in digital medical technology have occurred if it comes to “making the invisible visible.” Especially in craniomaxillofacial surgery, complex reconstructive procedures in primary or secondary cases have always been a challenge in the past and will remain as such in the future. Patients have a different view on their pathology than the surgeon has: the patient has an outside-oriented perspective, which relates to outer appearance and appropriate function. The surgeon has to take the outer tissue envelope into consideration to focus on the inside-oriented perspective, which is the craniofacial skeleton, and the associated soft tissues to analyze the amount of individual deformity, to modify these, and to finally achieve an appropriate esthetic result for that patient combined with adequate function.
Detailed knowledge of the anatomic structures is fundamental for orbital surgery: inadequate reconstructions potentially lead to unsatisfactory results. For this reason, clinical assessment, combined with imaging techniques used for diagnosis and treatment planning, must meet the highest standards. It is only then possible to answer the most important question in orbital fracture management: do we have to explore an orbital defect? This ongoing debate is further discussed in Leander Dubois and colleagues’ article,” Ongoing Debate in Clinical Decision Making in Orbital Fractures: Indications, Timing, and Biomaterials ,” in this issue. If surgery is indicated, it is the task of the surgeon to minimize the risk for the patient and achieve the adequate result, which brings the following questions: do we have a plan for our intended result? Is this plan achievable? Is the plan adequate? Is the plan available during surgery? Can feedback on the plan be obtained intraoperatively? Did we achieve the intended result?
These questions led to the introduction and implementation of computer-assisted surgery. From this perspective, all phases of treatment (before, during, and after the surgical procedure) should be optimized for the individual patient. Medical technology turned out to be the cornerstone to take this idea to reality: with technological developments, the limitations in orbital reconstruction could be overcome and the predictability of the results improved. This article covers the intraoperative phase of computer-assisted surgery; with the “why” provided, focus is on the past developments that led to intraoperative control, the current state of the art, and the possible future of feedback and quality control in orbital surgery: the how, the now, and the wow.
How: the history of intraoperative feedback
Historically, several 2-dimensional (2D) radiographs had been used to approach orbital deformity correction. Today none of these have any impact anymore, and—by the way—there never could be a plan nor a control to what was loaded in and on the orbit. Surgery of the orbit based on 2D radiographs must have been a walk in the mist and darkness. The headway to 3D imaging, in case of orbital reconstruction helical and cone-beam computed tomography (CBCT) (only in selected cases combined with MRI), meets the requirement to treat a 3D defect with the aid of a 3D data set. However, in the eighties of the last decade these data were only used to look at, which meant that trying to understand the individual patient’s deformity depended on the views selected by the radiologist. From the nineties onwards, computer technology allowed the surgeon to interactively use the volume data sets on independent analyzing platforms. Clinicians became able to modify the individual volume data set to the intended result.
Mirroring the unaffected side onto the deformed side was the first attempt to virtually correct the bony framework and create a digital blueprint for the reconstruction in unilateral craniofacial defects ( Fig. 1 ). This technique was developed and taken into routine by the Freiburg group of Rainer Schmelzeisen, Alexander Schramm, and Nils-Claudius Gellrich, in cooperation with the former Leibinger Company, trying to make the STN Zeiss/Leibinger navigation system applicable for craniomaxillofacial surgery in those days. All planning had to be performed on the navigation machine itself; it was only in the early 2000s that medical industry was able to serve the demands of the surgeon, and virtual planning became feasible with software on stand-alone machines, independent of the navigation system. It was the cooperation with Brainlab that resulted in this advancement in planning logistics and the availability of advanced software tools for virtual planning.

The navigation technology may be viewed as the offspring of frame-based stereotaxy, introduced by Horsley and Clarke in 1908. Their Horsley-Clarke apparatus, of which the first was produced at a cost of £300, included the use of geometric measurements in a Cartesian coordinate frame. , An atlas of the position of brain structures within the coordinate frame was generated for cats and monkeys, and the frame and atlas could even be compensated for different head sizes. It was not until 1947 that a modified version of the apparatus, proposed by Spiegel and Wycis, was used in human brain surgery. The link with the preoperative imaging was made with the introduction of frameless stereotaxy in 1987: the position of the pointer tip of an articulating mechanical arm was related to the image data acquired before the operation. , Optical tracking of the instrument and patient meant increased freedom of movement and easier use of the navigation system. The use of navigation in orbital surgery, almost a decade after Horsley and Clarke laid the groundwork, was kick-started with an article describing the use in a group of 18 patients by the Freiburg group. An image of one of the navigation systems used in these days is provided in Fig. 2 .

Developments in 3D imaging have had another effect next to the development of surgical planning and the utilization during surgical navigation: 3D imaging technology became available in the OR itself. Already in the early 1980s applications of imaging during neurosurgery were described, but the fixed position of the scanner and limited image quality hampered it widespread use. Advances in image quality, image equipment, and mobility of intraoperative CT or CBCT scanners over the years paved the way for utilization within oral and maxillofacial surgery: it is not surprising that reconstruction of orbital defects was among the first applications.
In the meantime, the gap had to be closed between virtual planning, navigation, and intraoperative imaging on one side and the implant to achieve the desired result on the other. Individualization of orbital implants was historically achieved by preshaping whatever biomaterial during surgery and was thereby depending on the experience and skills of the individual surgeon. Deeply respected colleagues such as Beat Hammer, Joe Gruss, Paul Manson, and others had their own way to prebend, or mold on a nonindividual reference model, with outstanding results. But even at that time they already included some kind of reference, whether it was schematic drawings only or the aforementioned reference skull draped with a sterile foil to serve as an aid for molding the reconstruction material. With further development of 3D printing technologies more and more individualized biomodels of the given (deformed) patient’s anatomy or the virtually corrected anatomy became available to shape a nonpreformed biomaterial toward an individual implant, either preoperatively or intraoperatively ( Fig. 3 ). , This technique still is a valid and an adequate method, which helps to master nearly all kind of craniofacial deformity correction. Latest technology allows to even provide 3D-printed patient-specific biomodels, which are autoclavable.

A different approach to shaping an implant was developed in parallel. Research on the average shape of the orbit performed by Marc Metzger’s group (Freiburg) was a preface for the development of a preformed orbital implant based on the average shape of the orbit and possible defects encountered. In theory, the use of such an implant prevents the necessity of preoperative or intraoperative bending altogether, thus saving time and being less dependent on the molding skills of the surgeon. , Another advantage lies in the possibility to import a virtual model of the preformed implant in the virtual surgical planning. The optimal position of the implant can be determined based on characteristics of patient and defect; it is even possible to evaluate preformed implants of several sizes or different manufacturers (see also article,“ Advanced Diagnostics and 3D Virtual Surgical Planning in Orbital Reconstruction ”, in this issue).
With emerging skills of analyzing patient’s volume data sets, the view of taking an individual virtual plan toward an adequate individual anatomic shaped implant changed the requirements of what an implant should be able to provide and represent: above the effect of an anatomic replacement the terms “functionalization” and “preventive design” of an implant came up. Functionalization implies that functions were added to the implant, for example, position control information was added for navigation purposes. Furthermore, anatomic extensions or flings can be added to an implant, so that the implant properly leavers on reliable structures in the defect site. Preventive design implies that typical mistakes that might occur with specific procedures can be diminished: in reconstructive orbital surgery this refers to change of implant shape, sharp edges, and possible interference with the optic nerve or eye muscles especially in the orbital cone area. This is why the Hannover Group with Majeed Rana and Nils-Claudius Gellrich developed a patient-specific orbital implant with a distinct topography of different thickness, a slightly thickened circular outer rounded cord-style implant border, an inverted snow-shovel design in the area of the posterior ledge, and multiple linear openings perpendicular to the transition zone for the medial orbital wall and perpendicular to the parasagittal oblique vector for the orbital floor. These implementations can be seen in Figs. 4 .

Now: current concepts in state-of-the-art quality control
Diagnosis precedes the computer-assisted reconstruction of the orbit and is based on clinical findings and 3D imaging. As discussed in article,” Advanced Diagnostics and 3D Virtual Surgical Planning in Orbital Reconstruction ”, in this issue CT must be regarded as the gold standard for primary diagnosis preceding orbital reconstruction and should be preferred. It provides not only excellent resolution of bone structures but, unlike CBCT, also allows soft-tissue structures to be evaluated, especially with the use of contrast agent. In current concepts of intraoperative feedback workflows, virtual surgical planning and simulation of the reconstruction serves as the reference of the feedback obtained. The objective of surgical planning is to perform an ideal reconstruction based on a virtual model that matches the desired outcome of surgery. , , ,
Although discussed in greater detail in article,” Advanced Diagnostics and 3D Virtual Surgical Planning in Orbital Reconstruction ”, in this issue several important factors include that many different types of simulations may be performed without a loss of information, 2D and 3D images may be displayed in any plane before and during surgery, and virtual models (STL data) may be imported. , These factors allow that standard implants, anatomically preformed implants, and patient-specific implants can be virtually positioned in the patient data set preoperatively and their shape, size, and fit can be assessed ( Fig. 5 ). , , If a patient-specific implant is indicated for the orbital reconstruction, the next step on the ladder is to process virtual reconstruction STL data sets using computer-aided design (CAD) software for planning patient-specific implants ( Fig. 6 A) and to directly produce these implants using computer-aided manufacturing (CAM) techniques such as selective laser melting ( Fig. 6 B). During the surgical procedure, surgeons have the difficult task of transferring the result of virtual computer-assisted planning to the surgical site as accurately as possible. For this purpose, they can use and combine a variety of different methods and devices, depending on the complexity of the task.

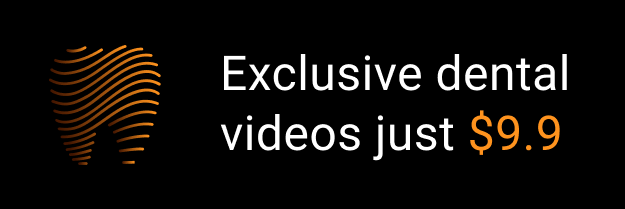