Introduction
The aim of this in-vitro study was to investigate the influence of cortical bone thickness on the amount of surface microdamage produced after insertion of orthodontic miniscrews (OM) in porcine tibia bone.
Methods
Aarhus OMs (Medicon, Tuttlingen, Germany; diameter, 1.5 mm; length, 6 mm) were inserted into 1.0 mm (group A; n = 10), 1.5 mm (group B; n = 10), and 2.0 mm (group C; n = 10) of porcine cortical bone using a torque-limiting hand screwdriver set at 18 Ncm. A sequential staining technique was used to identify microdamage under laser confocal microscopy. Virtual slices were stitched together using ImageJ software (National Institutes of Health, Bethesda, Md) to form a compressed 2-dimensional composition of the microdamage. The ImageJ software was used to quantify the total damage area, diffuse damage area, maximum crack length, maximum damage radius, and maximum diffuse damage radius. Kruskal-Wallis tests and Wilcoxon rank sum tests were used to analyze the data.
Results
All OMs in group A (1.0 mm) were inserted completely; however, 2 OMs from group B (1.5 mm) and all OMs in group C (2.0 mm) failed to insert completely. The entry surface of group C (2.0 mm) exhibited significantly higher amounts of total damage, diffuse damage area, maximum crack length, and maximum crack damage radius compared with groups A (1.0 mm) and B (1.5 mm). The maximum crack length observed on the entry and exit surfaces ranged from 1.03 to 3.06 mm.
Conclusions
In this study, we demonstrated a higher level of microdamage after the insertion of OMs into 2.0-mm thick cortical bone compared with 1.0-mm thick cortical bone. Therefore, clinicians need to consider the thickness of the cortical bone at the insertion site, because mechanisms to reduce cortical bone thickness would likely reduce the amount of microdamage formed. A safety zone of 3.5 mm from the OM is also recommended for OMs inserted into 1.0- and 1.5-mm cortical bone thicknesses to minimize any detrimental effects after targeted remodeling.
Highlights
- •
Cortical bone thickness affects the amount of microdamage after miniscrew insertion.
- •
Significant microdamage was seen when miniscrews were placed in 2-mm thick bone.
- •
Less microdamage occurred when bone was 1 or 1.5 mm thick.
- •
Clinicians should consider cortical bone thickness at the insertion site.
- •
A 3.5-mm safety zone should be used for miniscrews in 1- to 1.5-mm thick bone.
The field of orthodontic biomechanics has increased dramatically since the introduction of orthodontic miniscrews (OMs). Tooth distalization, intrusion, and the orthodontic camouflage of large skeletal discrepancies are now possible, particularly when dependence on patient compliance or anchorage from the dental arch is limited. OMs have proven to be an effective tool for patients with high anchorage demands. Subsequently, stability of the OM is vital to the success of the orthodontic treatment.
The stability of OMs has been studied extensively over the past few years, and failure rates between 0.0% and 40.8% have been documented. The variability and inconsistency of OM success are clinically unacceptable, and the unintentional loss of anchorage can negatively impact the orthodontic treatment outcome. Initial OM stability predominantly depends on primary stability, which describes the mechanical engagement and the relationship between the OM threads and surrounding bone immediately after insertion. The interlocking contact, however, elicits extensive mechanical deformation in the form of diffuse damage and distinct linear microcracks in the bone immediately adjacent to the OM. The amount of microdamage has been strongly associated with the initiation of site-specific bone remodeling and, accordingly, may result in a reduction of bone-implant contact and OM dislodgement. Minimizing the formation of microdamage might be an important determinant related to the clinical success of the OM.
OM success has been previously associated with procedure-related measures, patient factors, and OM characteristics, all of which could influence microdamage formed during insertion. Cortical bone thickness is considered a critical determinant in obtaining primary stability due to its strong correlation with pull-out strength and association with insertion torque. In the maxilla and the mandible, 2 mm apical to the alveolar crest, an appreciable fluctuation in cortical bone thickness, ranging from 0.85 to 2.58 mm, has been reported. Although OM insertion sites depend on access, surrounding anatomic landmarks, and orthodontic biomechanics, placement sites with cortical bone thicknesses greater than 1 mm have been shown to provide sufficient stability, whereas thinner cortical bone has been associated with OM failure and dislodgement. Results from finite element modeling appear to support these clinical findings by showing an increase in von Mises stress distribution and potential microdamage associated with thin cortical bone at insertion sites. Histologically, however, Wawrzinek et al reported no correlation between cortical bone thickness and the extent of microdamage caused during OM insertion.
Although the biomechanical analysis model lends support to the clinical observations, few histologic studies have been conducted to verify the correlation between microdamage and OM stability. Therefore, the aim of this study was to investigate the influence of cortical bone thickness on the amount of microdamage caused immediately after insertion of OMs. The null hypothesis was that cortical bone thickness would have no influence on the amount of microdamage after insertion. See Supplemental Materials for a short video presentation about this study.
Material and methods
Three porcine tibia bones were obtained from a local abattoir after approval from the Animal Ethics Committee (number M-2015-032) of the University of Adelaide. The proximal and distal ends of the bones were removed with a bandsaw, and the specimens were sectioned into quarters. Before use, the specimens were carefully stripped of attached soft tissues. A total of 30 bone specimens were machined under irrigation, using a slow-speed diamond saw, measuring 15 by 20 mm wide with nominal thicknesses of 1.0, 1.5, and 2.0 mm. The bone specimens were divided into 3 groups of 10 based on cortical bone thickness: group A (1.0 mm), group B (1.5 mm), and group C (2.0 mm). The specimens were polished with increasing grades of silicon carbide micromesh paper to remove any iatrogenic damage caused during the machining process. Subsequently, the thickness of each bone specimen was measured using digital calipers. A scratch was placed in the lower left corner of each specimen to differentiate the entry surface from the exit surface of the cortical bone. The prepared specimens were wrapped in gauze soaked with phosphate-buffered saline solution and stored at −20°C to maintain adequate hydration and preserve the physical properties of the bone until testing. A total of 30 self-drilling Aarhus Anchor screws (Medicon, Tuttlingen, Germany; 1.5 × 6.0 mm) were used.
At the time of testing, the specimens were immersed in xylenol orange (5 × 10 -4 mol/L) solution for 30 minutes to identify residual iatrogenic damage. The xylenol-stained specimens were rinsed thoroughly under distilled water for 8 minutes and then placed into a custom-made device designed to secure the bone and measure the compressive force applied during insertion. The customized rig incorporated an Omega miniature compression load cell, with a range of 0 to 100 N (R4-F6-76535; N2Surplus, Roanoke, Va), connected to a personal computer with LabVIEW software (National Instruments Australia, Macquarie Park, New South Wales, Australia) to digitize the compressive force ( Fig 1 ). The OMs were inserted manually into the bone specimens by 1 clinician (M.V.N.), using a torque-limiting hand driver (G00234; Rocky Mountain Orthodontics, Denver, Colo) with the torque limit set to 18 Ncm. The compression force data obtained from the LabVIEW software were transferred to Excel (Microsoft, Redmond, Wash) to determine the insertion consistency between the 3 groups.

After insertion, the specimens were submerged in a solution of calcein (1 × 10 -3 mol/L) for 30 minutes, followed by an 8-minute rinse under distilled water, to identify damage caused during miniscrew insertion. The specimens were then returned to the customized device for removal of the OMs. Any displaced bony segments protruding from the insertion or exit surface were removed with a scalpel to provide a more ideal imaging surface. Figure 2 shows the displaced bony segments formed after insertion. The specimens were immersed in calcein blue (1 × 10 -4 mol/L) solution for 30 minutes, to identify iatrogenic damage caused during removal of any gross bony debris and then rinsed in distilled water for 8 minutes.

Both the entry and exit surfaces of the bone specimens were imaged at 63 times magnification using a spectral scanning confocal microscope (SP5; Leica, Wetzlar, Germany). A 561DPSS laser (569-700 nm red spectrum), a 488 argon laser (496-593 nm green spectrum), and a 405 diode laser (413-480 nm blue spectrum) were used for the excitation and detection of xylenol orange, calcein, and calcein blue, respectively. A series of 10-μm virtual slices were generated to a maximum depth of 500 μm from the entry and exit surface. The images were stitched together using ImageJ (National Institutes of Health, Bethesda, Md) to form a compressed 2-dimensional composition of the microdamage ( Fig 3 ).

The microdamage on the entry and exit surfaces was quantified into regions of diffuse damage and linear microcracks. Diffuse damage was defined as regions of small cracks, measuring 1 μm, in a crosshatched appearance with intense halo staining. Microcracks, conversely, were identified as intermediate stained sharp linear defects in the order of 100 μm. The following histomorphometric measurements were made on the stitched images using the ImageJ program: total damage area, diffuse damage area, maximum crack length, maximum damage radius, and maximum diffuse damage radius ( Fig 4 ). Quantification analysis was performed on 10 randomly selected images 1 month after the initial histomorphometric measurements were taken, to determine intrarater reliability. In addition, another clinician analyzed 10 randomly selected images to determine the interrater reliability of the histomorphometric analysis.

Statistical analysis
Descriptive analyses were calculated for the specimens using software (version 9.3; SAS Institute, Cary, NC). Intraclass correlation coefficient analysis was used to determine intrarater and interrater reliability. Kruskal-Wallis tests and Wilcoxon rank sum tests were performed to investigate the differences in histomorphometric effects of cortical bone thickness on microdamage formation.
Results
Cortical bone thickness in groups A, B, and C did not differ significantly from 1.0, 1.5, and 2.0 mm, with mean values of 1.06 mm (SD, 0.06), 1.51 mm (SD, 0.08), and 1.96 mm (SD, 0.08), respectively. Intraclass correlation coefficient analysis indicated excellent intrarater and interrater reliabilities, ranging from 0.99 to 0.91 and 0.99 to 0.76, respectively.
Data from the compression load cell showed some variations between the 3 groups, despite implementation of the insertion protocol by 1 clinician. The discrepancy, however, can be attributed to the partial and complete insertions of the OMs. All OMs in group A (1.0 mm) were inserted completely, and partial insertion was achieved for 2 OMs in group B (1.5 mm) and all OMs in group C (2.0 mm), when insertion torque was limited to 18 Ncm. Partial insertion was defined as the inability of the OM collar to achieve bone contact. All partially inserted OMs displayed 5 exposed threads above the entry surface. Figure 2 , D , shows complete insertion of an OM from group A (1.0 mm), and Figure 2 , E , shows the same from group B (1.5 mm); Figure 2 , F , shows partial insertion of an OM from group C (2.0 mm).
The amount of observed microdamage increased with an increase in cortical bone thickness. Figure 5 shows the microdamage formed after insertion into 1.0-mm (A), 1.5-mm (B), and 2.0-mm (C) thick cortical bone. The entry surface of group C (2.0 mm) displayed statistically higher measurements for total damage area ( P <0.05), diffuse damage area ( P <0.05), maximum crack length ( P <0.05), and maximum damage radius ( P <0.05) compared with the entry surfaces of groups A (1.0 mm) and B (1.5 mm) ( Fig 6 ). Conversely, the exit surfaces only demonstrated a significantly higher value for maximum crack length for group C (2.0 mm) compared with groups A (1.0 mm) and B (1.5 mm) ( P <0.05) ( Fig 7 ).



When the microdamage on the entry surface was compared with the exit surface damage, the maximum crack length ( P <0.05) and the maximum damage radius ( P <0.05) consistently demonstrated significantly higher measurements across all 3 groups for the exit surface ( Table ).
Total damage area (mm 2 ) | Diffuse damage area (mm 2 ) | Maximum crack length (mm) | Maximum damage radius (mm) | Maximum diffuse damage radius (mm) | ||||||
---|---|---|---|---|---|---|---|---|---|---|
Mean | SD | Mean | SD | Mean | SD | Mean | SD | Mean | SD | |
Group A | ||||||||||
Side | ||||||||||
Entry | 7.58 | 0.95 | 6.59 | 0.75 | 1.12 | 0.50 | 2.37 | 0.40 | 1.87 | 0.28 |
Exit | 9.92 | 3.62 | 5.97 | 0.92 | 2.47 | 1.10 | 3.34 | 1.10 | 1.68 | 0.33 |
P value | 0.23 | 0.16 | <0.05 | <0.05 | 0.34 | |||||
Group B | ||||||||||
Side | ||||||||||
Entry | 8.55 | 1.22 | 7.42 | 0.89 | 1.24 | 0.49 | 2.39 | 0.35 | 1.95 | 0.19 |
Exit | 10.26 | 1.87 | 7.28 | 1.48 | 2.07 | 0.54 | 3.09 | 0.50 | 1.94 | 0.33 |
P value | <0.05 | 0.79 | <0.05 | <0.05 | 0.47 | |||||
Group C | ||||||||||
Side | ||||||||||
Entry | 10.81 | 1.07 | 8.64 | 0.99 | 2.07 | 0.29 | 3.14 | 0.47 | 2.03 | 0.21 |
Exit | 9.37 | 2.71 | 6.11 | 1.66 | 3.15 | 0.90 | 3.85 | 0.80 | 1.89 | 0.28 |
P value | 0.39 | <0.05 | <0.05 | 0.054 | 0.24 |
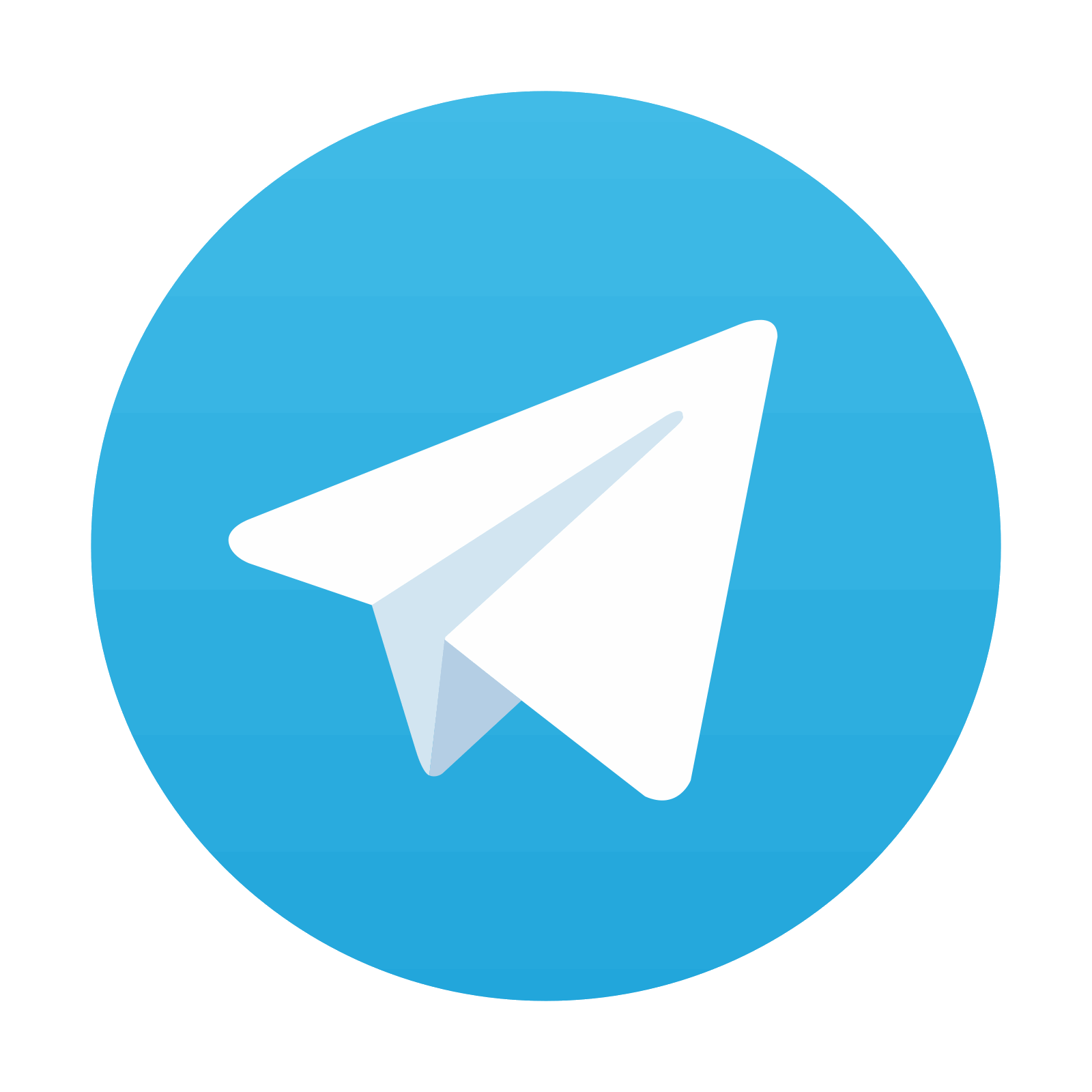
Stay updated, free dental videos. Join our Telegram channel

VIDEdental - Online dental courses
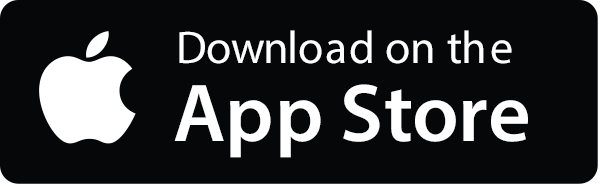
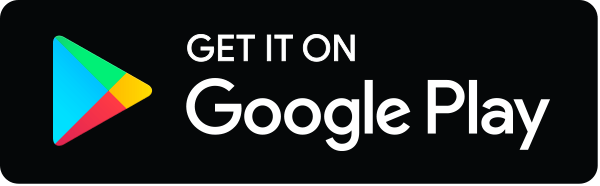
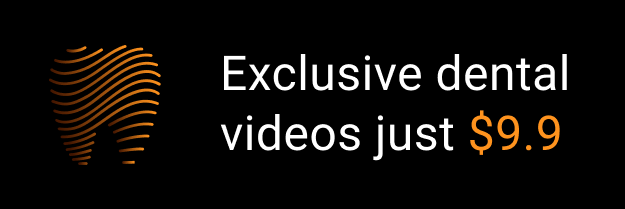